Abstract
Microalgae and microalgae-derived ingredients are one of the top trends in the food industry. However, consumers’ acceptance and purchase intention of a product will be largely affected by odour and flavour. Surprisingly, the scientific literature present a very limited number of studies on the volatile composition of microalgae and cyanobacteria. In order to fill the gap, the main objective of the present study was to elucidate the volatile composition of seven microalgal and cyanobacterial strains from marine and freshwaters, with interest for the food industry while establishing its potential impact in odour. Among the seven selected strains, Arthrospira platensis showed the highest abundance and chemical diversity of volatile organic compounds (VOCs). Aldehydes, ketones, and alcohols were the families with the highest diversity of individual compounds, except in Arthrospira platensis and Scenedesmus almeriensis that showed a profile dominated by branched hydrocarbons. Marine strains presented a higher abundance of sulfur compounds than freshwater strains, while the ketones individual profile seemed to be more related to the taxonomical domain. The results of this study indicate that the VOCs composition is mainly driven by the individual strain although some volatile profile characteristics could be influenced by both environmental and taxonomical factors.
Similar content being viewed by others
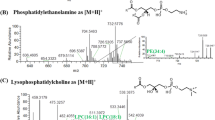
Introduction
Microalgae and cyanobacteria are being mass cultured for a wide range of industrial applications, however, most of the microalgal biomass is currently being used for the manufacture of food supplements (rich in protein, carotenoids, or polyunsaturated fatty acids)1. Arthrospira maxima and Arthrospira platensis (AP) are the most common strains of cyanobacteria used in food applications (commercialised as Spirulina). The microalga Chlorella vulgaris (CV) is also widely used in the food industry, mainly in Asia2. Other species from the genus Nannochloropsis, Scenedesmus, Haematococcus, Dunaliella, or Tetraselmis have also been used for the manufacture of food supplements at lab-scale1. In addition, the microalga Tetraselmis chuii has been authorised as a novel food in accordance with Regulation (EC) No 258/973.
Microalgae and microalgae-derived ingredients are one of the top trends in the food industry, and the amount of microalgae-containing foods launched into the marked has steadily increased during the last decade, being this trend likely to continue to grow1,4. Among the main constrains related to the use of microalgae in food industry, there are some related with their production such as high production costs and low yields5, but also others related with quality such as their (generally) green colour and strong marine taste and odour6,7. Odour and flavour are of key importance as both will largely affect the consumers’ acceptance or rejection of a product as well as the purchase intention. In the scientific literature, there is a very limited number of studies on the volatile composition of microalgae and cyanobacteria, and to the best of the authors’ knowledge, there are no studies on the relationship between volatile profile and their origin (marine or freshwater). Among the few studies on the matter, sulfur compounds, esters, and alcohols have been identified in the biomass of Crypthecodinium cohnii while aldehydes and alcohols were detected in Schizochytrium limacinum8. Several volatile compounds identified in seafood such as sulfur compounds (dimethyl disulphide, dimethyl trisulphide, and methional), diketones (2,3-butanedione, 2,3-pentadione and 2,3-octanedione), and terpenoids (α- and β-ionone) were identified in different microalgal strains namely Botryococcus braunii, Rhodomonas, Chlorella, Tetraselmis, and Nannochloropsis8,9.
The aim of the present study is to determine the volatile composition and potential odour impact of seven strains of microalgae and cyanobacteria from marine and freshwater analysed by solid-phase microextraction followed by gas chromatography coupled to mass spectrometry.
Results and discussion
Identification and semi-quantitative information of the individual VOCs found in the microalgal and cyanobacterial strains are listed in Table 1. Results listed by chemical family are presented in Table 2.
Overall, 150 volatile compounds were detected in the seven strains studied (Table 1). The number of detected VOCs described in previous bibliography is quite variable. Van Durme et al.9 detected 57 compounds in five microalgal strains (Botryococcus braunii, Rhodomonas, Tetraselmis sp., Nannochloropsis oculata and Chlorella vulgaris), Zhou et al.10 described 246 compounds in six microalgal strains during three growth phases (Thalassiosira weissflogii, Nitzschia closterium, Chaetoceros calcitrans, Platymonas helgolandica, Nannochloropsis sp. and Dicrateria inornata), and de Jesus et al.11 detected only 29 compounds in AP. This wide variation in the number of identified compounds may be due to the low number of the studies reported to date and differences in the methodologies used for volatile extraction and analysis.
Total number of VOCs varied significantly among strains being AP and Scenedesmus almeriensis (SA) the richest strains with 95 and 102 VOCs respectively. Isochrysis galbana (IG) (81), CV (78), Tetraselmis sp. (TS) (78), Nannochloropsis gaditana (NG) (63), and Synechococcus sp. (SY) (52) presented lower VOCs diversity (Table 2). These results highlighted the high variability in both individual volatile compounds and chemical families among different microalgae. Results were in line with those described by Zhou et al.10 who observed variations ranging between 46 and 84 compounds depending on microalgal strain and growth phase. In terms of relative abundance (RA), IG and AP were the richest species (P ≤ 0.05) for total VOC abundance (Table 1). To the best of the authors’ knowledge, this is the first report describing the volatile profile of SA, SY, and IG. As a whole, the main chemical families in terms of number of compounds for all strains analysed were branched hydrocarbons (40), aldehydes (18) and ketones (17) (Table 2). These three chemical families have been previously reported as major chemical classes in microalgae9,10.
Aldehydes
IG and CV were the richest species in both number of aldehydes and percentage of RA (17 compounds and 18.2%, and 16 compounds 21.7%, respectively; Table 2). The number of acyclic aldehydes was very variable between the different strains (between 3 and 18 compounds). In turn, only 3 cyclic aldehydes were detected, being their percentage of relative abundance only relevant in TS (3.7%). Only shortchain aldehydes (between 6 and 9 carbons) were found in the selected strains probably due to the low extraction temperature used (30 ºC)12. In terms of RA, the microalgal strain with the highest content (P ≤ 0.05) of acyclic aldehydes was IG followed by AP and CV (Table 1). Again, AP showed a fairly extraordinary situation with very few aldehydes detected and a very high content of propanal, 2-methyl-, 2-pentenal, 2-methyl-, hexanal, and 2-butenal, 2-methyl- were the most abundant aldehydes in IG, while the RA of hexanal was five times higher than that of any other aldehyde in CV as reported in previous studies9. Particularly noteworthy was the high RA of 2,4-heptadienal, (E,E)- in SY. Among cyclic aldehydes, benzaldehyde was the major compound in all species with the exception of AP that was very rich in β-cyclocitral (Table 1).
Short-chain aldehydes (alkanals, alkenals, and alkadienals) are formed from fatty acid autoxidation (mainly long-chain fatty acids with more than 18 carbon atoms)13. On the other hand, benzaldehyde and butanal, 3-methyl- have been related with amino acid degradation9,14, and β-cyclocitral together with β-ionone are derived from enzymatic degradation of β-carotene15. Aldehydes can contribute to microalgal and cyanobacterial odour because most of them show low odour threshold (OT) values (Table 3). Overall, saturated aldehydes have been related with green-like and hay-like odour notes whereas unsaturated aldehydes can impart fatty and oily odours9. Butanal, 3-methyl-, hexanal, heptanal, 2-octenal, (E)-, and β-cyclocitral were the aldehydes with higher odour impact values in most of the strains while propanal, 2-methyl- was the most important odorant exclusively in AP. β-Cyclocitral has been identified as a powerful odorant and one of the most abundant VOCs in cyanobacteria16. It was found in all species except for NG and SY, as previously reported9.
Hydrocarbons
A total of 12 linear, 4 aromatic, 40 branched, and 5 alicyclic hydrocarbons were identified in the studied microalgal and cyanobacterial strains. These hydrocarbons showed a moderated variation in both the number of individual compounds and percentage of relative abundance among strains. The most remarkable variability was in branched hydrocarbons which only occurred in two freshwater species (SA and AP). These species presented 40 and 39 branched compounds, respectively, whereas the maximum number detected in the other species ranged between 2 and 7 individual compounds. Similarly, branched hydrocarbons represented 2–9% of total VOCs relative abundance in most strains, although the abundance of these compounds in SA and AP was much higher (P ≤ 0.05) (46.2 and 37.3% of total VOCs, respectively; Table 2). It is important to highlight that, in the present work, some branched hydrocarbons were only identified to chemical family level due to the similarity among mass spectra and linear retention index (LRI) between branched compounds, the limited bibliographic information on LRI values, and the scarce availability of commercial high purity compounds. This noteworthy VOCs present in AP was overlooked in the scarce bibliography available, despite the presence of branched hydrocarbons have been highlighted in superior algae17 such as Capsosiphon fluvescens18 and Undaria pinnatifid 19 or even in other microalgae such as Nostoc sp.20. On the other hand, it is also remarkable the similarity of the VOCs profile of AP and SA, even though SA is a eukaryotic microalga and AP a prokaryotic cyanobacterium (Fig. 1). The individual chromatographic VOCs profile of each strain is provided as Supplementary Table S1. Among individual compounds, a larger proportion of large linear hydrocarbons (more than 13 carbon atoms) were found in prokaryotic cyanobacteria (Table 1) as reported previously16. In this respect, some of these linear hydrocarbons could have contaminated the samples during the production and freeze-drying process of strains in the laboratory, or during storage of the samples in plastic containers.
The origin of branched hydrocarbons has been previously related with the oxidation of branched-chain fatty acids in other food matrices21. However, it should be noted that in some microalgae species such as AP, very low branched-chain fatty acid contents have been reported22. Therefore, it is likely that these compounds are coming from a secondary route, but further research is needed to understand the origin of these compounds. In general, acyclic hydrocarbons have been described to have no significant flavour contribution in food matrices13 and it was confirmed by the odour impact ratio (OIR) values estimated in the selected strains (Table 3). Moreover, the contribution of branched hydrocarbons to flavour is still quite uncertain as there are no OT values available for these compounds in specialised databases or the scientific literature.
Aromatic hydrocarbons can be assumed to be generated mainly from the degradation of aromatic amino acids23. They are usually known as important aromatic compounds. However, in the present work, the only one that seemed to impact in a moderate way (OIR values from 168 to 655) was benzene, ethenyl- (Table 3).
Ketones
Acyclic (18) and cyclic (8) ketones were deemed a very representative chemical class in some of the studied strains. In this regard, IG and CV were the microalgae with the highest number of individual acyclic ketones (15 each) and also the highest percentage of RA (P ≤ 0.05) (15.9 and 13.9%, respectively). In turn, cyclic ketones were more representative of the VOCs profile in TS, SA, and AP in both number of compounds and percentage of RA (Table 2). In macroalgae or seaweed, acyclic ketones are usually related with less evolved brown seaweeds, while cyclic ketones are related with more evolved species19. In the current study, cyanobacterial strains showed a lower number of cyclic ketones than most microalgae (Table 2). The relative abundance of acyclic ketones was significantly higher in IG when compared to the other strains (Table 1), mainly due to the extremely high abundance of 3,5-octadien-2-one (E,E)- and (Z,Z)- in the former. Both configuration isomers were also present as major ketones in SA and CV and they were detected exclusively in eukaryote microalgae whereas among prokaryote strains, the major ketone was 2-propanone (Table 1). Van Durme et al.9 reported 1-penten-3-one, 3-pentanone and 2-butanone, 3-methyl- as major ketones in microalgae species. In addition, acyclic ketones were very similar between marine and freshwater strains in both, relative abundance and number of individual compounds.
Branched cyclohexanones and cyclopentanones were major compounds among cyclic ketones in all the studied strains. Cyclohexanone, 2,2,6-trimethyl- has been previously reported as a characteristic ketone in cyanobacteria23. Isophorone was present in both cyanobacteria and 4-oxoisophorone was a major ketone in CV and SA. Furthermore, 1,3-cyclopentanedione, 2-methyl- showed significantly (P ≤ 0.05) higher RA in IG, TS, and SA than in the other strains. In line with the high relative abundance (P ≤ 0.05) of β-ionone found in AP, cyanobacteria were previously featured as a rich source of carotenoids23. However, the results of the present work indicated that the presence of nor-carotenoids was strain-dependent, since β-ionone was not detected in the SY.
The origin of acyclic ketones is variable, while linear ketones are derived from lipid oxidation, some methyl ketones may result from β-oxidation of the fatty acids and subsequent decarboxylation and others are mainly products of oxidative cleavage of carotenoids as above-mentioned for β-cyclocitral25. In general, acyclic ketones are related with desirable odours in food. Saturated ketones are related with sweet, floral, and fruity odour notes, while unsaturated ketones are responsible for green odour notes10. Moreover, Van Durme et al.9 related the seafood-like odour in microalgae to their high content in diketones such as 2,3-pentanedione and 3,5-octadien-2-one. In the present work, these compounds were found in considerable amounts in microalgae, while the only diketone found in cyanobacteria was 3,5-heptadien-2-ona, 6-methyl- (exclusively in AP). Prokaryotic chlorophytes such as AP have been previously related with high amounts of methyl-ketones such as 2-propanone, 2-pentanone, 2-peptanone and 2-octanone which contribute to green odour notes24. Attending to OIR values (Table 3), acyclic ketones generated a great odour impact mainly in IG and AP. 2,3-Pentanedione, 2-heptanone, 6-methyl-, and 3,5-octadien-2-one (E,E)- presented the highest OIR values in IG while 2-heptanone, 6-methyl- was also deemed important in AP. The latter compound has been included in the camphoreous odour family (Supplementary Table S1). Among cyclic ketones, β-ionone was the major odorant in freshwater strains, and one of the most abundant odorants in marine strains (Table 3). Previous studies reported β-ionone as a potent odorant in some microalgae such as Scenedesmus sp. and CV and also in macroalgae25,26. In addition, this compound has been related to the characteristic odour of microalgae10,25 together with β-ionone, 5,6-epoxy-.
Alcohols
Alcohols in microalgae are mainly formed as secondary decomposition of hydroperoxides of fatty acids, with the exception of branched alcohols that may also derive from carbohydrates via glycolysis or from amino acids through the Ehrlich pathway27. In the present study, marine eukaryotes IG, NG, and TS were the strains presenting higher diversity of acyclic alcohols with 9, 8, and 8 individual compounds detected respectively. The number of cyclic alcohols found in these species was lower and similar between them (Table 2). Similarly, the percentage of relative abundance of acyclic alcohols was, in general, significantly higher in marine (11.2–18.6%) than in freshwater strains (7.2–15.6%), except for CV that presented a high proportion of acyclic alcohols (15.6%). Overall, the abundance in cyanobacteria was significantly smaller (0.65–5.55%) when compared with microalgal strains. The diversity of cyclic alcohols was similar among the studied strains and TS presented the highest proportion (P ≤ 0.05) of cyclic alcohols (7.48%) (Table 2). Regarding individual compounds, 1-penten-3-ol, 2-penten-1-ol, (Z)-, and 1-octen-3-ol were major compounds in all eukaryote strains while very different alcohol composition was observed between prokaryotes. Briefly, SY showed negligible amounts of both acyclic and cyclic alcohols in comparison to the other strains (P ≤ 0.05) whereas major alcohols in AP were 1-hexanol, 2-hexen-1-ol, (Z)- and cyclohexanol, 2,4-dimethyl-. It is remarkable the absence of geosmin and isoborneol, 2-methyl- in freshwater microalgae and specially in cyanobacterial strains since these two compounds have been previously reported as odorant compounds related to earthy-muddy odour notes in cyanobacterial24. Commercial high purity standards of both compounds were analysed in order to ensure their absence in cyanobacteria strains. These results were comparable to those of previous reports in which geosmin and isoborneol, 2-methyl- were no detected in AP16 nor in other freshwater species9. Results reported herein were in line with previous research on VOCs content in microalgae, where TS presented lower content of alcohols when compared to CV and NG (Table 1)9. In contrast, Van Durme et al.9 found low amounts of 1-octen-3-ol while in the present study this acyclic alcohol was major in most of the studied strains as in dehydrated edible seaweed19. Although alcohols have relatively high OT values, some unsaturated alcohols may exert an important impact in odour20. In the present work, 1-octen-3-ol (earthy, green, oily, fungal, grassy, and fatty) was deemed as one of the compounds with higher OIR in most species (Table 3).
Nitrogen and sulfur containing compounds
Nitrogen- and sulfur-containing compounds were also detected in the headspace of the selected strains. Sulfur compounds were deemed as major compounds (P ≤ 0.05) (in terms of relative abundance; Table 1) in marine microalgae, particularly in IG and NG where they represented 29.6 and 37.5% of total VOCs, respectively (Table 2). In turn, sulfur compounds were not detected, or detected in negligible abundance, in cyanobacteria and freshwater strains. The most abundant sulfur compound in microalgae was methyl sulfide, although the abundance of dimethyl sulfoxide was also important in some species such as IG (Table 1). Nitrogen compounds abundance was lower than that of other chemical families and only in IG, TS, SA and AP reached percentages between 3 and 5% of total VOCs abundance (Table 2).
The formation of sulfur compounds has been previously related with catabolism of free, peptide and protein sulfur-containing amino acids28, while in marine algae the presence of dimethyl sulfide was related to the degradation of sulfonio propionate, dimethyl-18. On the other hand, nitrogen compounds are commonly described as derived from Maillard reactions due to high temperatures applied to food or biological matrices27,28, and pyrazines and pyridines have been reported as abundant in dried seaweeds29. In the present work, the microalgal strains were dehydrated by freeze-drying and the temperature used for volatile extraction was low (30 ºC). Therefore, it is unlikely that those procedures could originate thermal-derived nitrogen compounds. Probably, the pyrazines found in the headspace of microalgae and cyanobacteria could be originated from trimethylamine N-oxide degradation during storage15.
Both nitrogen- and sulfur-containing compounds have been related to fish odours10,13. Sulfur compounds were associated with the characteristic aroma of marine crustaceous13 while alkylpyrazines generally deliver roasty odour notes28 and nutty odour such as the case of pyrazine, methyl- (Supplementary Table S1). In fish, this compound has been related with fishy and ammonia odours. Nitrogen compounds seem to contribute little to the final odour due to their low OIR values (lower than 100) in most species (Table 3). On the contrary, the low OT values for methyl sulfide together with its high abundance in marine microalgae strains make methyl sulfide the most important odorant in these species (Table 3). In this regard, previous studies have reported that sulfur compounds were responsible for characteristic odours of marine microalgae such as cooked shrimp/cooked seafood and marine and fishy odours9.
Esters, furans and other compounds
The RA of the esters in the strains was small and each individual compound seemed to be characteristic of each specie. Ethyl and methyl acetate were previously reported in microalgae biomass10. Diethyl phthalate was found in all the studied strains, being phthalate products regarded as toxic pollutants18.
Furans were found as constant compound present in microalgae VOC composition. The number of furan compounds was very similar in both marine and freshwater strains although a significantly (P ≤ 0.05) higher percentage of RA was observed in CV (9.00%) in comparison with the rest of the species (Table 2). The abundance of furan 2-methyl-, furan, 2-pentyl-, and furan, 2-ethyl- was particularly considerable in IG, TS, and CV (Table 1). Moreover, furans have been reported previously as microalgae VOCs9,16. Furans can be formed by Amadori pathways from the oxidation of fatty acids or by glucose pyrolysis28. In general, furans have been identified as off-flavours of fat and oils imparting a beany, grassy liquorice, and tobacco odour notes27.
Materials and methods
Microalgae and cyanobacteria strains selection and production
Selected strains included Isochrysis galbana (IG—REC 0002B), Nannochloropsis gaditana (NG—BEA 1202), Tetraselmis sp. (TS—BEA 0098/2), Chlorella vulgaris (CV—CCAP 1475/9), Synechococcus sp. (SY—PCC 7942), Arthrospira platensis (AP—BEA 0005B), and Scenedesmus almeriensis (SA—CCAP 276/24), which is a lutein overproducing strain isolated by the Chemical Engineering Department of the University of Almería (Spain). Selected strains were produced in controlled closed bubble column photobioreactors located inside a greenhouse at the pilot plant facilities of the University of Almería. Full taxonomic characteristics of the strains used in the present work are provided in Fig. 1.
Daily maximum, minimum, and average temperature inside the greenhouse were 27.0 ± 2.2, 11.7 ± 1.7 and 18.4 ± 1.7 °C, respectively. Average irradiance during the approximately 12 h of sunlight was 600.2 ± 72.2 µE/m2·s with peaks of 1500–1600 µE/m2·s at midday. The pH of all the strains except for AP was controlled by on-demand injection of carbon dioxide at 8.0. Culture media used for the production of CV and SA was the Arnon medium30 for AP the Arnon medium supplemented with sodium bicarbonate (16.8 g/L; pH 9.5 ± 0.2) and for NG, TS, IG and SY the Algal medium31. Once the biomass concentration reached approximately 1.5 g/L, the biomass was harvested and concentrated by centrifugation using a Sigma 3–18 KS centrifuge (Sigma Laborzentrifugen, Osterode am Harz, Germany) operating at 8000g for 10 min. The concentrated biomass with a concentration of approximately 20 g/L was immediately frozen at −80 ºC and freeze-dried using a Crydos-50 freeze-dryer (Telstar, Barcelona, Spain). The obtained dried powder was stored in a sealed plastic container at room temperature until further analysis.
Solid-phase microextraction of volatile compounds
Detailed description of the chemicals and suppliers used in the present experiment are described in the Supplementary Data.
Freeze dried microalgal/cyanobacterial biomass was weighted (0.300 ± 0.001 g) in triplicate in 10 mL amber vials (Agilent Technologies, Madrid, Spain) and 10 μL internal standard (IS) were added (0.1 mg/mL of cyclohexanone in hexane solution). Vials were subsequently sealed with PTFE septa and a steel magnetic cap (18 mm PTFE/SIL, Agilent Technologies), vortexed for 15 s, and left in a chilled room (4 ± 1 ºC) for 24 h prior to analysis.
The solid-phase microextraction procedure was performed using a PAL RSI 85 autosampler (CTC Analytics, Zwingen, Switzerland). After 15 min of pre-equilibration time at the extraction temperature, volatile compounds were trapped onto a 1 cm long divinylbenzene/carboxen/polydimethylsiloxane fiber (57298-U, 50/30 µm, Supelco, Madrid, Spain) at 30 ºC for 30 min.
Volatile compounds trapped onto the fiber were desorbed in the front injection port of the GC equipment for 15 min at 240 °C in splitless mode (split valve was opened at 200 mL/min after 10 min of the injection) using the autosampler device. After thermal desorption, the fiber was directly cleaned in the back injection port for 30 min at 270 °C.
The working routine of the automatic sampler was to perform a blank (empty 10 mL amber vial) every three sample analyses. All the microalgal and cyanobacterial samples were analysed on the same day, and the samples were randomly located in the autosampler tray.
Gas chromatography–mass spectrometry analysis
Volatile compounds were analysed using a 7820A gas chromatograph (Agilent Technologies) equipped with two split/splitless injectors and coupled to a 5975 series mass spectrometry detector (Agilent Technologies). The volatile compounds were separated in a Supelcowax-10 (Supelco) fused silica capillary column (60 m long, 0.25 mm i.d., 25 µm film thickness) as described in Moran et al.12.The mass spectrometer consisted in a single quadrupole operating in full scan mode (1.4 scans/s, m/z range 26–350) at 230 ºC with a total ion current of 70 eV.
Chromatographic data were analysed with MSD ChemStation Data Analysis (version 5.52, Agilent Technologies). The limit of detection (LOD) was calculated from the noise obtained in the analysis of ten blanks. LOD was set as twice the average noise for each chromatographic zone. Mean linear retention index (LRI) values were calculated using the average real retention time of three replicates of each compound and the retention time of the standard saturated alkanes certified reference material. LRI values showed a variation coefficient less than 0.15% for all individual volatile compounds.
Tentative identification of volatile compounds was performed by comparing their mass spectra (matching factor > 800) with those of the National Institute of Standards and Technology (NIST version 2.0, Gaithersburg, USA). Additionally, peak identifications were confirmed by comparison of experimental LRI values with those previously published for volatile compounds analysed under similar chromatographic conditions when available. Positive identification was performed by comparison of the experimental LRI and mass spectra with those of commercial standards. Chromatographic peak areas were measured using selective integration for the four more abundant m/z ions of each target compound according to NIST mass spectra.
Peak areas (> LOD) of individual volatile compounds detected in at least two of the three replicates were used to calculate mean abundances in each sample. The volatile compound content of the samples was expressed as relative abundance (RA, arbitrary area units) to the area of IS according to the following equation:
Peak areas were multiplied by 10–5 for easier comprehension and three significant figures were used to express the RA of volatile compounds in the samples.
Odour impact ratio of volatile compounds
The odour intensity of the different volatile compounds identified was estimated by means of the odour impact ratio (OIR). Briefly, available odour threshold (OT) values measured in water were collected from available databases32,33,34, and the OIR for the individual volatile compounds was calculated as follows:
Additionally, odour notes for volatile compounds were described according to The Good Scents Company database35 and Giri et al.27,28 and are provided in Supplementary Table S1.
Statistical analysis
Statistical analysis of data was performed using IBM-SPSS version 25.0 (IBM, Armonk, USA). One-way analysis of variance (ANOVA) was applied to determine the statistical significance of the differences in the volatile composition of microalgae and cyanobacteria individual species. Levene’s test was used to verify data homoscedasticity. Tukey’s test was used for pairwise comparison among individual species. When a variable was not homoscedastic, the robust Welch test was applied, and Games-Howell test was used for pairwise comparisons. In case of lack of normality of the variables for both individual RA and percentage of RA of chemical families, the non-parametric Kruskal–Wallis H test was applied. Statistical significance was declared at P ≤ 0.05.
Conclusions
Microalgae and cyanobacteria are rich in VOCs and their characteristic volatile profile is strongly strain-dependent. Prokaryotic cyanobacteria, generally included within the term microalgae, are less prone to generate acyclic diketones which have been related to fishy odour notes when compared with eukaryotic microalgae. However, the influence of the individual species on the volatile profile was very significant since AP and SY cyanobacteria species showed completely different volatile profiles. Sulfur compounds can be considered as characteristic volatile compounds in marine microalgae, whereas some freshwater species such as AP and SA are rich in branched hydrocarbons. Results presented herein indicate that the volatile profile of microalgae should be individually evaluated since this profile is strongly strain-dependent. Further research is needed to relate the volatile profile with the biochemical and other compositional characteristics of each specie. Assessing the organoleptic attributes of microalgae (and cyanobacteria) is important when used for food applications, as the marine flavour and odour attributed to many microalgae strains could be used as a strategy to potentiate culinary preparations or develop novel innovative foods.
References
Lafarga, T. Cultured microalgae and compounds derived thereof for food applications: Strain selection and cultivation, drying, and processing strategies. Food Rev. Int. 36(6), 559–583. https://doi.org/10.1080/87559129.2019.1655572 (2020).
Lafarga, T., Fernández-Sevilla, J. M., González-López, C. & Acién-Fernández, F. G. Spirulina for the food and functional food industries. Food Res. Int. 137, 109356. https://doi.org/10.1016/j.foodres.2020.109356 (2020).
AECOSAN. Report of the Scientific Committee of the Spanish Agency for Consumer Affairs, Food Safety and Nutrition (AECOSAN) on a request for initial assessment for marketing of the dried marine microalgae Tetraselmis chuii in food supplements under Regulation (EC) No 258/97 on novel foods and novel food ingredients. in Revista del Comité Científico de la AECOSAN, 25, 11–21. AECOSAN, Ministerio de Sanidad, Servicios Sociales e Igualdad, Madrid, Spain (2017).
Koyande, A. K. et al. Microalgae: A potential alternative to health supplementation for humans. Food Sci. Hum. Welln. 8(1), 16–24. https://doi.org/10.1016/j.fshw.2019.03.001 (2019).
Garrido-Cardenas, J. A., Manzano-Agugliaro, F., Acien-Fernandez, F. G. & Molina-Grima, E. Microalgae research worldwide. Algal Res. 35, 50–60. https://doi.org/10.1016/j.algal.2018.08.005 (2018).
Chacón-Lee, T. L. & González-Mariño, G. E. Microalgae for “healthy” foods—Possibilities and challenges. Comprehens. Rev. Food Sci. Food Saf. 9(6), 655–675. https://doi.org/10.1111/j.1541-4337.2010.00132.x (2010).
Lafarga, T. Effect of microalgal biomass incorporation into foods: Nutritional and sensorial attributes of the end products. Algal Res. 41, 101566. https://doi.org/10.1016/j.algal.2019.101566 (2019).
Hosoglu, M. I. Aroma characterization of five microalgae species using solid-phase microextraction and gas chromatography–mass spectrometry/olfactometry. Food Chem. 240, 1210–1218. https://doi.org/10.1016/j.foodchem.2017.08.052 (2018).
Van Durme, J., Goiris, K., De Winne, A., De Cooman, L. & Muylaert, K. Evaluation of the volatile composition and sensory properties of five species of microalgae. J. Agric. Food Chem. 61(46), 10881–10890. https://doi.org/10.1021/jf403112k (2013).
Zhou, L. et al. Change of volatile components in six microalgae with different growth phases. J. Sci. Food Agric. 97(3), 761–769. https://doi.org/10.1002/jsfa.7794 (2017).
de Jesus, C. S. et al. Outdoor pilot-scale cultivation of Spirulina sp. LEB-18 in different geographic locations for evaluating its growth and chemical composition. Biores. Technol. 256, 86–94. https://doi.org/10.1016/j.biortech.2018.01.149 (2018).
Moran, L., Aldai, N. & Barron, L. J. R. Elucidating the combined effect of sample preparation and solid-phase microextraction conditions on the volatile composition of cooked meat analyzed by capillary gas chromatography coupled with mass spectrometry. Food Chem. 352, 129380. https://doi.org/10.1016/j.foodchem.2021.129380 (2021).
Lindsay, R. C. Flavour of Fish. Seafoods: Chemistry, Processing Technology and Quality 75–84 (Springer, 1994).
Cremer, D. R. & Eichner, K. The reaction kinetics for the formation of Strecker aldehydes in low moisture model systems and in plant powders. Food Chem. 71(1), 37–43. https://doi.org/10.1016/S0308-8146(00)00122-9 (2000).
Kawai, T. & Sakaguchi, M. Fish flavor. Crit. Rev. Food Sci. Nutr. 36(3), 257–298. https://doi.org/10.1080/10408399609527725 (1996).
Milovanović, I. et al. Determination of volatile organic compounds in selected strains of cyanobacteria. J. Chem. https://doi.org/10.1155/2015/969542 (2015).
López-Pérez, O., Picon, A. & Nuñez, M. Volatile compounds and odour characteristics of seven species of dehydrated edible seaweeds. Food Res. Int. 99, 1002–1010. https://doi.org/10.1016/j.foodres.2016.12.013 (2017).
Sun, S., Chung, G. & Shin, T. Volatile compounds of the green alga, Capsosiphon fulvescens. J. Appl. Phycol. 24(5), 1003–1013. https://doi.org/10.1007/s10811-011-9724-x (2012).
Vilar, E. G., O’Sullivan, M. G., Kerry, J. P. & Kilcawley, K. N. Volatile compounds of six species of edible seaweed: A review. Algal Res. 45, 101740. https://doi.org/10.1016/j.algal.2019.101740 (2020).
Dembitsky, V. M., Shkrob, I. & Dor, I. Separation and identification of hydrocarbons and other volatile compounds from cultured blue-green alga Nostoc sp. by gas chromatography–mass spectrometry using serially coupled capillary columns with consecutive nonpolar and semipolar stationary phases. J. Chromatogr. A 862(2), 221–229. https://doi.org/10.1016/S0021-9673(99)00930-9 (1999).
García, C. et al. Volatile components of dry cured Iberian ham. Food Chem. 41(1), 23–32. https://doi.org/10.1016/0308-8146(91)90128-B (1991).
Bujard, E., Baco, U., Mauron, J., Mottu, F., Nabholtz, A., Wuhrmann, J. J., & Clément, G. Composition and nutritive value of blue green algae (Spirulina) and their possible use in food formulations. in Paper Presented at the 3rd International Congress of Food Science and Technology, Washington D.C. (1970).
Schulz, S. & Dickschat, J. S. Bacterial volatiles: The smell of small organisms. Nat. Prod. Rep. 24(4), 814–842. https://doi.org/10.1039/B507392H (2007).
Watson, S. B. Cyanobacterial and eukaryotic algal odour compounds: Signals or by-products? A review of their biological activity. Phycologia 42(4), 332–350. https://doi.org/10.2216/i0031-8884-42-4-332.1 (2003).
Rzama, A., Benharref, A., Arreguy, B. & Dufourc, E. J. Volatile compounds of green microalgae grown on reused waste water. Phytochemistry 38(6), 1375–1379. https://doi.org/10.1016/0031-9422(94)00835-H (1995).
Yamamoto, M. et al. Determination of volatile compounds in four commercial samples of Japanese green algae using solid phase microextraction gas chromatography mass spectrometry. Sci. World J. https://doi.org/10.1155/2014/289780 (2014).
Giri, A., Osako, K., Okamoto, A. & Ohshima, T. Olfactometric characterization of aroma active compounds in fermented fish paste in comparison with fish sauce, fermented soy paste and sauce products. Food Res. Int. 43(4), 1027–1040. https://doi.org/10.1016/j.foodres.2010.01.012 (2010).
Giri, A., Osako, K. & Ohshima, T. Identification and characterisation of headspace volatiles of fish miso, a Japanese fish meat based fermented paste, with special emphasis on effect of fish species and meat washing. Food Chem. 120(2), 621–631. https://doi.org/10.1016/j.foodchem.2009.10.036 (2010).
López-Pérez, O., del Olmo, A., Picon, A. & Nuñez, M. Volatile compounds and odour characteristics of five edible seaweeds preserved by high pressure processing: Changes during refrigerated storage. Algal Res. 53, 102137. https://doi.org/10.1016/j.algal.2020.102137 (2021).
del Mar Morales-Amaral, M., Gómez-Serrano, C., Acién, F. G., Fernández-Sevilla, J. M. & Molina-Grima, E. Outdoor production of Scenedesmus sp. in thin-layer and raceway reactors using centrate from anaerobic digestion as the sole nutrient source. Algal Res. 12, 99–108. https://doi.org/10.1016/j.algal.2015.08.020 (2015).
Ippoliti, D. et al. Modeling of photosynthesis and respiration rate for Isochrysis galbana (T-Iso) and its influence on the production of this strain. Biores. Technol. 203, 71–79. https://doi.org/10.1016/j.biortech.2015.12.050 (2016).
Burdock, G. A. Fenaroli’s Handbook of Flavor Ingredients 6th edn. (CRC Press, 2009).
Leffingwell & Associates. Odor & Flavor Detection Thresholds in Water (In Parts per Billion). http://www.leffingwell.com/odorthre.htm. Accessed 5 May 2021 (2020).
The Good Scent Company. The Good Scents Company Information System. http://www.thegoodscentscompany.com. Accessed 5 May 5 2021 (2021).
Van Gemert, L. J. Odour Thresholds: Compilations of Odour Threshold Values in Air Water and Other Media 2011th edn. (Oliemans Punter & Partners BV. Zeists, 2011).
Buttery, R. & Ling, L. 2-Ethyl-3,5-dimethylpyrazine and 2-ethyl-3,6-dimethylpyrazine: Odor thresholds in water solution Lwt-Food Sci. Technol. 30, 109–110. https://doi.org/10.1006/FSTL.1996.0139 (1997).
Plotto, A., Margaria, C., Goodner, K., Goodrich, R. & Baldwin, E. Odour and flavour thresholds for key aroma components in an orange juice matrix: terpenes and aldehydes. Flavour Fragrance J. 19, 491–498. https://doi.org/10.1002/FFJ.1470 (2004).
Czerny, M., Christlbauer, M., Christlbauer, M. & Fischer, A. Re-investigation on odour thresholds of key food aroma compounds and development of an aroma language based on odour qualities of defined aqueous odorant solutions. Eur. Food Res. Technol. 228(2), 265–273. https://doi.org/10.1007/s00217-008-0931-x (2008).
Fabrellas, C., Matia, L. & Ventura, F. Determination of odour threshold concentrations and dose-response relations in water of several minor disinfection by-products: aldehydes and alkyl nitriles. Water Sci. Technol. 49(9), 267–272. https://doi.org/10.2166/wst.2004.0585 (2004).
Rashash, D. M. C., Dietrich, A. M. & Hoehn, R. C. Flavor profile analysis of selected odorous compounds. J. Am. Water Works Assoc. 89(2), 131–142 (1997).
Schnabel, K. O., Belitz, H. D. & Von Ranson, C. Investigations on the structure-activity relation of odorous substances. I. Detection thresholds and odor qualities of aliphatic and alicyclic compounds containing oxygen functions. Z. Lebensmittel Untersuchung Forschung 187, 215–223 (1988).
Masuda, H. & Mihara, S. Olfactive properties of alkylpyrazines and 3-substituted 2-alkylpyrazines. J. Agric. Food Chem. 36(3), 584–587. https://doi.org/10.1021/jf00081a044 (1988).
Tandon, K. S., Baldwin, E. A. & Shewfelt, R. L. Aroma perception of individual volatile compounds in fresh tomatoes (Lycopersicon esculentum, Mill.) as affected by the medium of evaluation. Postharvest. Biol. Technol. 20, 261–268. https://doi.org/10.1016/S0925-5214(00)00143-5 (2000).
Mayer, F. & Grosch, W. Aroma simulation on the basis of the odourant composition of roasted coffee headspace. Flavour Fragance J. 16, 180–190. https://doi.org/10.1002/ffj.975 (2001).
Young, W. F., Horth, H., Crane, R., Ogden, T. & Arnott, M. Taste and odour threshold concentrations of potential potable water contaminants. Water Res. 30(2), 331–340. https://doi.org/10.1016/0043-1354(95)00173-5 (1996).
Tamura, H., Boonbumrung, S., Yoshizawa, T. & Varanyanond, W. The volatile constituents in the peel and pulp of a green thai mango, khieo sawoei cultivar (Mangifera indica L.). Food Sci. Technol. Res. 7(1), 72–77. https://doi.org/10.3136/fstr.7.72 (2001).
Buttery, R. G. & Ling, L. C. Volatile flavor components of corn tortillas and related products. J. Agric. Food Chem. 43(7), 1878–1882. https://doi.org/10.1021/jf00055a023 (1995).
Takeoka, G. R., Flath, R. A., Mon, T. R., Teranishi, R. & Guentert, M. Volatile constituents of apricot (Prunus armeniaca). J. Agric. Food Chem. 38(2), 471–477. https://doi.org/10.1021/jf00092a031 (1990).
Pino, J. A. & Mesa, J. Contribution of volatile compounds to mango (Mangifera indica L.) aroma. Flavour Fragrance J. 21, 207–213. https://doi.org/10.1002/ffj.1703 (2006).
Boonbumrung, S. et al. Characteristic aroma components of the volatile oil of yellow keaw mango fruits determined by limited odor unit method. Food Sci. Technol. Res. 7(3), 200–206 (2001).
Zoetman, B. C. J., Kraayeveld, A. J. A. & Piet, C. J. Oil pollution and drinking water odors. Water 4(16), 367–371 (1971).
Acknowledgements
Tomas Lafarga thanks the Spanish Ministry of Science, Innovation, and Universities (IJC2018-035287-I) and the BBVA Foundation. L. Moran contract was funded by the program for research groups of excellence of the Basque Government (IT944-16).
Funding
This work was conducted in the framework of the SABANA Project funded by the EU H2020 Research and Innovation Programme (Grant agreement 727874), ALGALPROT Project funded by CEI·MAR (CEIJ-002) and financial support provided by the Basque Country Government (IT944-16).
Author information
Authors and Affiliations
Contributions
L.M. Conceptualization, Methodology, Validation, Formal analysis, Investigation, Writing—original draft, Writing—review & editing; G.B. Formal analysis, Investigation; N.A. Investigation, Writing—review & editing; M.C. Investigation; A.M.E. Investigation; A.S.Z. Investigation; L.J.R.B. Methodology, Formal analysis, Resources, Supervision, Writing—Review & Editing, Project administration, Funding acquisition; T.L. Supervision, Writing—Review & Editing, Funding.
Corresponding author
Ethics declarations
Competing interests
The authors declare no competing interests.
Additional information
Publisher's note
Springer Nature remains neutral with regard to jurisdictional claims in published maps and institutional affiliations.
Supplementary Information
Rights and permissions
Open Access This article is licensed under a Creative Commons Attribution 4.0 International License, which permits use, sharing, adaptation, distribution and reproduction in any medium or format, as long as you give appropriate credit to the original author(s) and the source, provide a link to the Creative Commons licence, and indicate if changes were made. The images or other third party material in this article are included in the article's Creative Commons licence, unless indicated otherwise in a credit line to the material. If material is not included in the article's Creative Commons licence and your intended use is not permitted by statutory regulation or exceeds the permitted use, you will need to obtain permission directly from the copyright holder. To view a copy of this licence, visit http://creativecommons.org/licenses/by/4.0/.
About this article
Cite this article
Moran, L., Bou, G., Aldai, N. et al. Characterisation of the volatile profile of microalgae and cyanobacteria using solid-phase microextraction followed by gas chromatography coupled to mass spectrometry. Sci Rep 12, 3661 (2022). https://doi.org/10.1038/s41598-022-07677-4
Received:
Accepted:
Published:
DOI: https://doi.org/10.1038/s41598-022-07677-4
Comments
By submitting a comment you agree to abide by our Terms and Community Guidelines. If you find something abusive or that does not comply with our terms or guidelines please flag it as inappropriate.