« Prev Next »
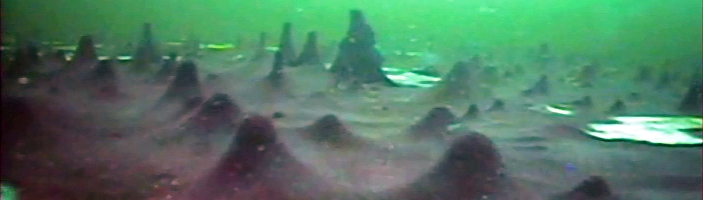
Imagine being able to look back into the distant past through a "time-portal." Wouldn't it be fascinating to see life as it was 2.5 billion years ago (bya) in the shallow seas of the early Earth? These were harsh times when there was no available oxygen, and life was entirely composed of microorganisms. Well, scientists have discovered such a place within collapsed depressions along the lake floor in what are called submerged sinkholes in Lake Huron, one of the five Great Lakes located in North America. The sinkholes' inhabitants — mostly microbes — are providing us a glimpse of what early life on Earth might have been like and how it might have transformed the planet. Here, colorful microbial mats with bubbling gasses have been discovered which are not found anywhere else in the Great Lakes, and are known to occur in just a few other places on Earth. Since 2002, scientists interested in the geology, hydrology, chemistry and biology of sinkholes have explored these habitats. Explorations using research vessels, remotely operated vehicles (ROVs), scuba divers, underwater sensors, metabolic chambers, sediment corers, as well as laboratory-based studies are revealing a fascinating working picture of this unique ecosystem (Biddanda et al. 2009; Figure 1a).
Characteristics of Lake Huron's Submerged Sinkholes
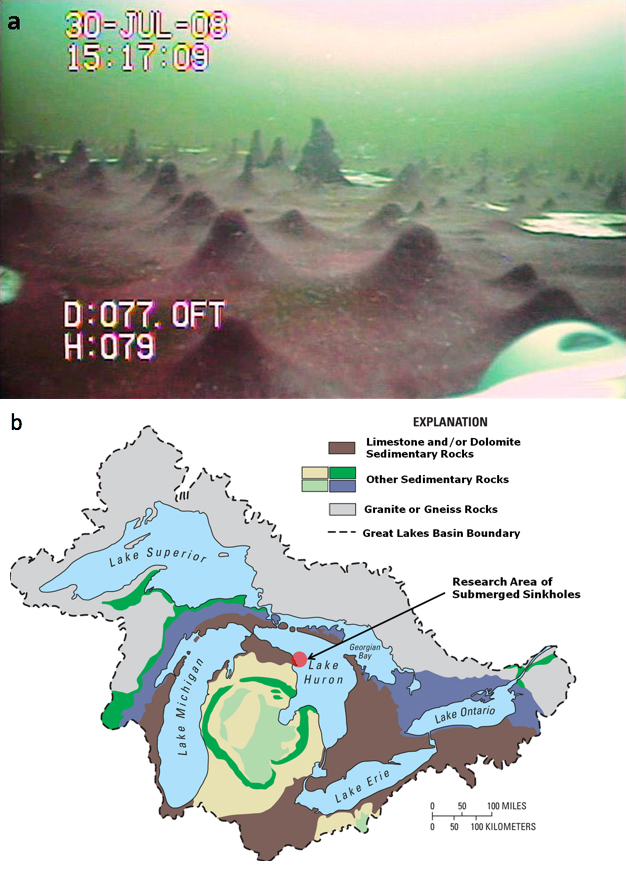

To understand the significance of life in underwater sinkholes, let's explore the sinkholes themselves. How were they formed? Lake Huron, the third largest of the Great Lakes, is contained within a basin underlain by ~400 million year old (0.4 bya) limestone, dolomite, and gypsum bedrock — rock that was formed from the remains of saltwater seas that once covered the continent (Figure 1b, Grannemann et al. 2000). Over time, movement of groundwater in and around these bedrocks gradually dissolved them, forming underground caves and cracks called karst formations (Figure 2a). Further erosion of bedrock along the cave ceilings caused collapses leaving visible sinkholes. Figure 2b shows areas of on-land sinkholes and submerged sinkholes around Alpena, Michigan. Here, we focus our discussion on a particular submerged sinkhole located 2 miles offshore in 23 m of water near Middle Island (Figures 2b and 3a).
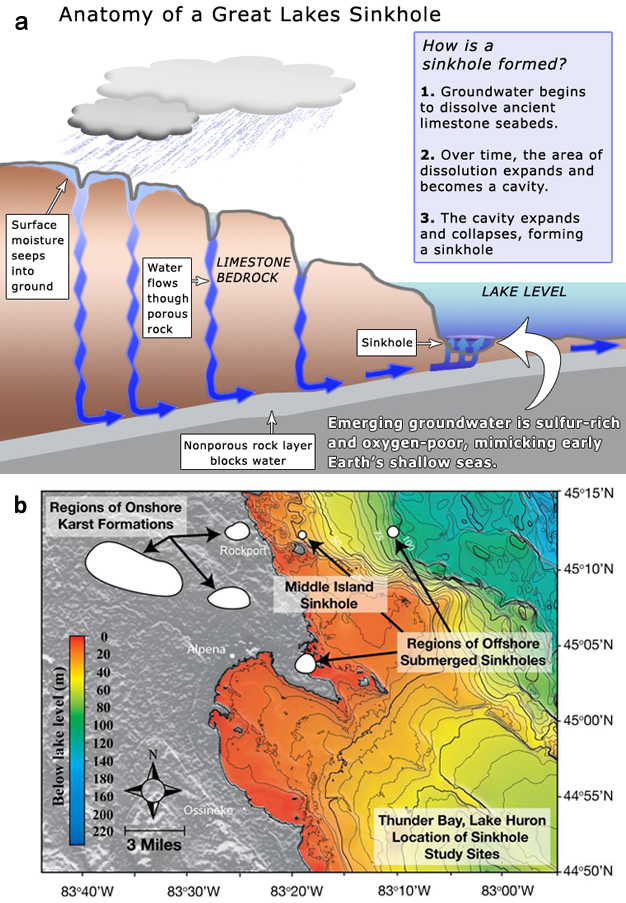

What are the characteristics of the venting groundwater that nurture extensive microbial growth (Figure 1)? To answer this question, we gathered data using a variety of water quality sensors, and collected samples of lakewater and groundwater for laboratory analyses. We found two important clues: venting groundwater contained no oxygen, but had high levels of the element sulfur, measured in the form of sulfates (Table 1, Figure 3b). Many microbes and higher organisms need oxygen for their survival, so the submerged sinkholes sustained by groundwater are an extremely different habitat when compared to normal lakes. The temperature of the venting groundwater keeps the sinkhole's floor at a stable and cool 9oC. The groundwater also had lower redox potential, pH, dissolved organic carbon, and nitrate levels, and higher dissolved inorganic carbon, chloride and carbonate ions. Higher level of ions is also indicated by the higher conductivity measured in the groundwater. These geochemicals are picked up as groundwater slowly dissolves the limestone, dolomite, and gypsum bedrock — also known as marine evaporite layers. In particular, erosion of gypsum deposits provides the groundwater with sulfate levels that are 100 times higher than Lake Huron water.
Parameter | Lake Huron | Venting GW |
Sp. Conductivity (mS/cm) | 0.2 | 2.3 |
Chloride (mg/L) | 6 | 25 |
Temperature (oC) | seasonal | ≈9 |
pH | 8.3 | 7.1 |
ORP (mV) | 500 | -134 |
Dissolved Oxygen (mg/L) | 11 | ≈0 |
Sulfate (mg/L) | 15 | 1250 |
DIC (mg/L) | 19 | 48 |
DOC (mg/L) | 1.7 | <1 |
Table 1. Comparison of physicochemical properties of Lake Huron water and venting Groundwater at Middle Island Sinkhole, Lake Huron (ORP = oxidation-reduction potential; DIC = dissolved inorganic carbon; DOC = dissolved organic carbon). |
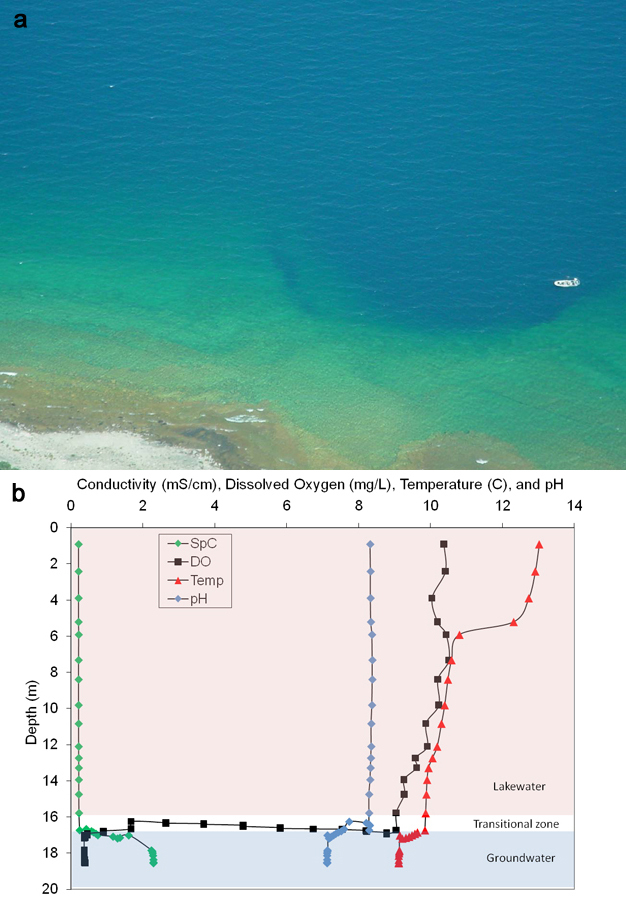

Because venting groundwater is cold, oxygen-poor, and ion-rich, it is denser than normal lake water, and forms a distinct layer on the lake floor that is as much as 5 m thick, or 22% of the overall water depth (Figure 3b). Eventually the layer thins out farther away from the groundwater source. Freshwater organisms, common to oxygenated Great Lakes habitats, cannot survive here. Instead, these conditions favor salt-loving, low oxygen-tolerant microbial mats that blanket the lake floor (Figure 1a). Microscopic, metabolic, and genetic studies show the mat-building organisms are primarily purple filamentous photosynthetic organisms, but also consist of white non-photosynthetic bacteria and other microbes. These mat microbes work in concert with microbes in the underlying sediment (see below).
A Bit of Background on Microbes in the Biogeosphere
Life is everywhere on Earth — in soil, air, ice and water — even in the most inhospitable environments. Microorganisms have been found thriving in superheated sediments deep beneath the ocean floor (Whitman et al. 1998) and within stones from the coldest and driest place on earth such as the dry valleys of Antarctica (Vestal 1988). Indeed, life has been found both 10 km above and 10 km beneath the Earth's surface (Schlesinger et al. 2011). This life-filled shell surrounding the Earth is known as the biosphere. Over time, the biosphere has shaped conditions on our planet, creating the oxygen in our atmosphere and storing massive amounts of carbon in coal and oil deposits. In turn, the biosphere has been shaped by the geosphere, or the rocky, inorganic materials of Earth's crust. Thus, the bio- and geo-spheres have co-evolved in a "biogeochemical tango" since Earth first formed ~4.6 bya.
Although exceptionally small, microorganisms dominate the biosphere (Whitman et al. 1998), and greatly impact the environment as a consequence of their daily activities — they are truly small players with very large roles (Cotner & Biddanda 2002). Indeed, tiny microbial engines drive the global cycles of carbon, oxygen, nitrogen, sulfur and other elements that are essential to all life (Falkowski et al. 2008). Over time, the collective might of their versatile physiologies has shaped Earth's atmosphere and changed the face of the planet (Kump 2008, Schlesinger et al. 2011). On early Earth, life formed under an atmosphere composed of nitrogen, carbon dioxide, and reduced gases such as hydrogen, methane, and ammonia — but no oxygen. Through photosynthesis, bacteria — and much later, green plants — excreted oxygen as a waste product, thereby creating the oxic atmosphere we now enjoy. Today, microorganisms mediate global cycling of carbon dioxide, oxygen and methane, and continue to act as key regulators of Earth's climate. Clearly, microorganisms have impacts vastly disproportionate to their small size, and represent a vast frontier awaiting exploration.
Microbes (Bacteria, Archaea and Eukarya) Dominate the Sinkhole Ecosystem
Much like early Earth, microorganisms dominate the Middle Island Sinkhole in Lake Huron. Scientists now recognize three domains, or superkingdoms, on the tree of life (Woese et al. 1990): the Bacteria, physiologically versatile, highly successful, and enormously diverse; the Archaea, renowned for their ability to thrive in extreme environments and perform unique physiologies such as methane generation; and Eukarya, the plants and animals (like us) as well as fungi and protists. DNA analysis determined that most of the purple mat microorganisms are of a strain of Phormidium autumnale, a photosynthetic cyanobacterium (Figure 4a,b). These long, filamentous organisms harvest light energy and create organic carbon from carbon dioxide (CO2) dissolved in the groundwater. Interestingly, Phormidium species are also found in such geographically remote locations as Yellowstone National Park (Wyoming, USA) and permanently ice covered lakes in Antarctica. We also found methane-producing Archaea deep within the sediment, and several Eukaryotic species that grow in the mat. While most of their DNA sequences are similar to known organisms, some are new sequences that may represent novel and undiscovered microbial life forms.
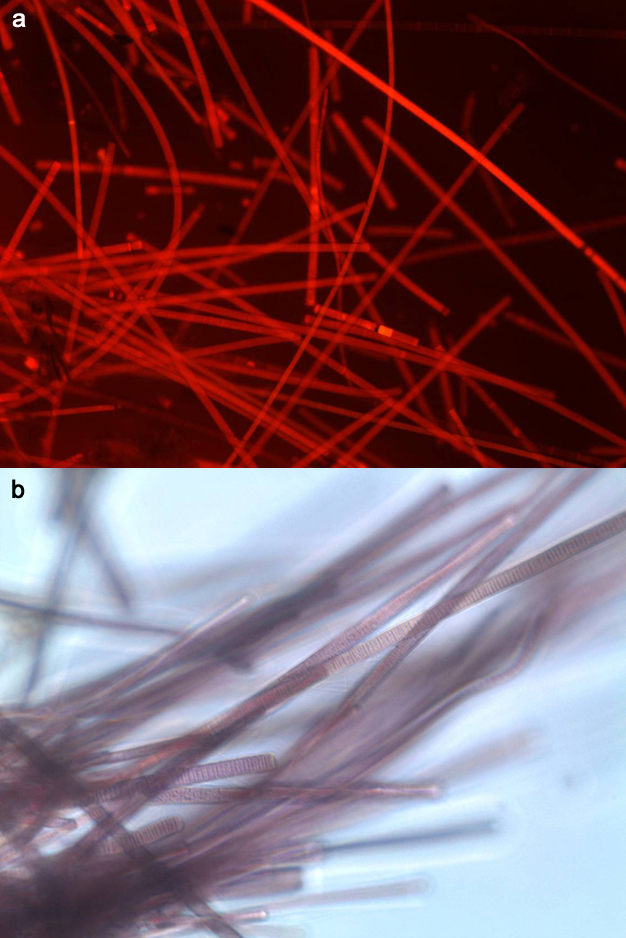

Sinkhole Mat Physiology: Working with Carbon and Sulfur Molecules
Sinkhole organisms are unique and diverse, but what functions do they perform? What are their similarities to early life? Sinkhole microorganisms constantly affect the lake environment by gathering and changing the forms of nutrients (Figure 5a). Each species of microbe has the capacity to perform specific metabolic or physiologic processes, but working with other microbial species, the mat community has developed capabilities that are quite amazing. In the submerged sinkholes, microbial mats have developed a partnership where some microbes convert the sulfate in the groundwater to sulfide. Then, other microbes convert the sulfide back to sulfate. Like on early Earth, the sinkhole microbes use the sulfur molecules for creating organic matter and obtaining energy.
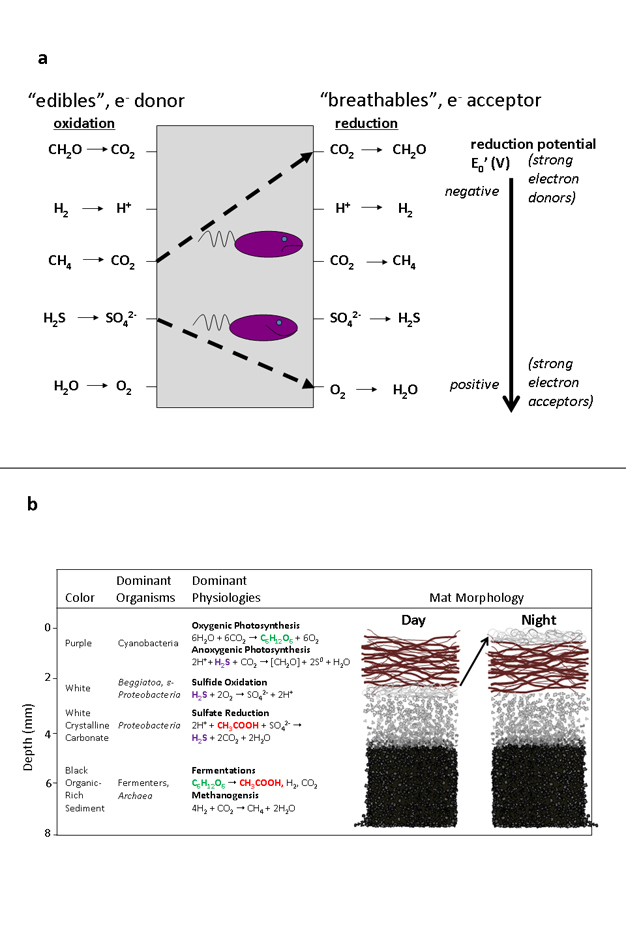

All living organisms use organic carbon to build cells and grow (Mix et al. 2006). Organisms (including humans) that obtain organic carbon from the environment are called heterotrophs, whereas those that can make their own organic matter (sugars) from inorganic carbon (carbon dioxide) are referred to as autotrophs or primary producers. Creating organic carbon from inorganic carbon is an energetic challenge, but sinkhole microbes have evolved three ways to do this by harnessing either solar or chemical energy (Figure 5b):
1) Oxygenic photosynthesis:
- Converts carbon dioxide and water into sugar and oxygen.
- Requires sunlight.
- Same process used by plants.
- Provides the oxygen we breathe.
2) Anoxygenic photosynthesis:
- Converts carbon dioxide and sulfide into sugar and sulfate.
- An ancient variant of photosynthesis that uses hydrogen sulfide instead of water as a source of electrons.
- Requires sunlight
- Does not produce oxygen.
3) Chemosynthesis:
- Converts carbon dioxide and reduced chemicals (e.g., sulfide) into sugar and oxidized minerals (e.g., sulfate).
- Instead of light energy, gathers the energy in inorganic chemicals such as sulfide.
- Does not use sunlight (i.e., can occur in the dark).
- Does not produce oxygen.
In the sinkholes, inorganic carbon is converted (fixed) into organic forms by all three mechanisms, and this organic matter is used for energy. During the process, microbes and nutrients (carbon and sulfur) cycle throughout the mat in a well-orchestrated dance that moves in step with day and night. The purple cyanobacteria glide upward during the day, reaching their filaments to soak up the energy in sunlight to fix carbon. Phormidium can perform both oxygenic and anoxygenic photosynthesis, switching between them depending on the availability of sulfide. During the night, white sulfur-oxidizing bacteria swarm over the purple cyanobacteria, and perform chemosynthesis by gathering sulfide and creating more fixed carbon.
Completing the Carbon and Sulfur Cycles
Microorganisms use a wide range of electron (e-) donors ("edibles" such as sugars) and electron acceptors ("breathables" such as oxygen) to generate energy for metabolism (Figure 5). Many of the production processes use sulfide (H2S), but groundwater only supplies them with sulfate (SO42-), so where does the sulfide come from? The sediment contains no oxygen, so bacteria living there must find alternative "breathables" — molecules that can accept electrons liberated during energy-yielding reactions (Figure 5a). Chemistry of sediment porewater suggests that sulfate is the dominant breathable in the habitat as explained by the simultaneous removal of sulfate and organic acids in the subsurface sediments (Nold et al. 2010). Sulfate reduction is a process that burns or oxidizes organic matter (organic acids like acetate) while reducing sulfate to sulfide. Sulfate-reducing bacteria are some of the earliest forms of life on Earth.
In the upper reaches of the mat, sulfate-reducing bacteria use edible fermentation products and breathable sulfate to make sulfide gas (in blue), a reactive form of sulfur. By locally raising the pH, sulfate-reducing bacteria also precipitate carbonate granules, resulting in a white mineral layer just beneath the cyanobacteria. Sulfide supplies power for further sulfur cycle reactions. During the day, sulfide donates electrons to anoxygenic photosynthesis performed by cyanobacteria (Figure 5b). Compare the two forms of photosynthesis, and notice how sulfide takes the place of water, forming sulfate instead of oxygen.During the night, sulfide supplies energy for sulfur oxidizing bacteria that combine it with oxygen, creating sulfuric acid as a by-product. There is no light for cyanobacteria to glide towards or use, so the sulfur oxidizing bacteria swarm over the mat surface, scrubbing oxygen from the overlying water while accessing sulfide diffusing up from below. Notice how sulfur is tightly cycled — sulfate reducing bacteria use the sulfate steadily supplied by cyanobacteria during the day and sulfur oxidizing bacteria at night. These sulfur physiologies are living analogs of activities performed long ago by fossil microbial mats that thrived in the oxygen-poor, sulfur-rich seas.
Fermentation and Methane Gas Production
Modern Sinkholes as Analogs of Proterozoic Seas (~2.5–0.5 bya)
What can sinkholes tell us about the past? Could modern day sinkhole microbes hold clues to the early oxygenation of the Earth? Sinkholes harbor the types of cyanobacteria that mediated dramatic global changes on ancient Earth, including perhaps the greatest turning point in the history of Earth and life, the oxygenation of the atmosphere. The "great oxidation event" (GOE) ~2.4 bya, set the stage for the evolution of complex life, including plants and animals (Figure 6). Cyanobacteria are thought to have driven the GOE by evolving oxygenic photosynthesis, a metabolism that consumes H2O and produces O2 as a waste product. Prior to the innovation of oxygenic photosynthesis, anoxygenic photosynthesis predominated — instead of being fueled by H2O, it is fueled by other electron donors such as H2 or H2S. The balance between these two forms of photosynthesis is determined by oxygen levels in the oceans and the atmosphere as follows. Anoxygenic photosynthesis (and chemosynthesis) is a net sink for oxygen because it fixes carbon without producing oxygen — much of this organic carbon will fuel microbial respiration that then consumes oxygen. On the other hand, oxygenic photosynthesis produces both organic carbon and oxygen-if some of the organic carbon gets buried through sedimentation, oxygen accumulates in the atmosphere.
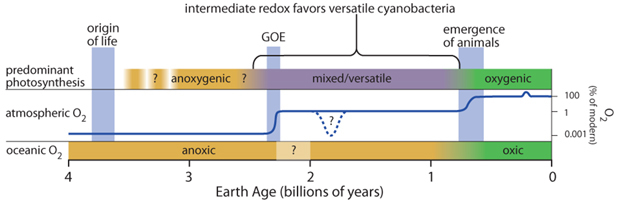

Conclusions and Future Prospects
Great Lakes sinkholes provide intriguing insights into life in Earth's history and allow us to "see" into our geochemical and metabolic origins. Interdisciplinary studies of Great Lakes sinkholes are uncovering new organisms and biochemical processes-leading to the conservation of these unique habitats and expanding our understanding of the immense diversity of life on Earth (Figure 7). According to the American Academy of Microbiology (2001), "Living creatures and the inanimate worlds they inhabit dance an intimate tango". A challenge for future students of nature will be to untangle the complex "biogeochemical tango" taking place within the varied ecosystems that constitute Earth's amazing biosphere. Additional information is available at http://gvsu.edu/wri/biddanda/learningcenter.
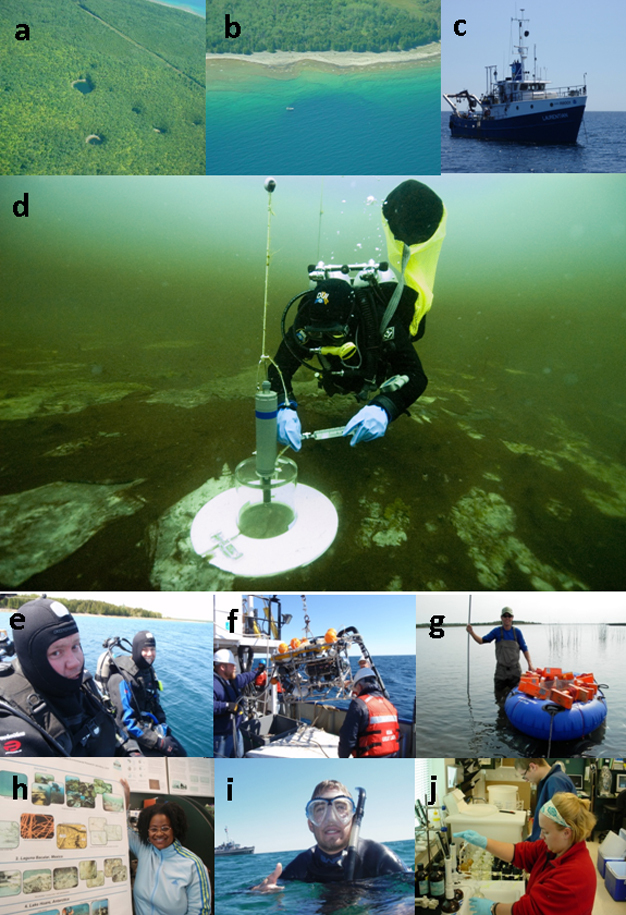

Acknowledgements:
Glossary
Anoxic: Containing no oxygen (O2).
Anoxygenic photosynthesis: Photosynthesis that does not produce O2 as a by-product.
Archaea: Domain of single-celled microorganisms often abundant in extreme environments.
Autotroph: An organism that produces organic carbon compounds from simple inorganic compounds using energy from sunlight (photosynthesis) or inorganic chemicals (chemosynthesis).
Bacteria: Domain of single-celled microorganisms ubiquitous on Earth.
Biosphere: The zone of life or the sum of all ecosystems on Earth.
Carbon cycle: Biogeochemical cycle by which carbon is exchanged among its different reservoirs on Earth.
Chemosynthesis: Process that converts carbon dioxide into organic compounds using the chemical energy from inorganic compounds (such as hydrogen sulfide), rather than sunlight. Can occur in light or dark.
Dolomite: Sedimentary rock composed of calcium magnesium carbonate.
Eukarya: Domain of both micro- and macro-organisms with membranous organelles like nucleus.
Gypsum: Sedimentary rock composed of calcium sulfate.
Heterotroph: An organism that cannot fix inorganic carbon, and therefore uses pre-formed organic carbon for growth.
Karst formations: Sedimentary carbonate rock (limestone, dolomite) that has been eroded by water.
Limestone: Sedimentary rock composed of calcium carbonate.
Microbe: Microorganisms too small to be seen by the human eye (0.02–20µm size) and composed of viruses, Bacteria, Archaea and smaller Eukarya.
Microbial mat: Filamentous microbes forming a cover on a surface, such as lake bottom.
Oxic: Containing oxygen (O2).
Oxygenic photosynthesis: Photosynthesis that produces O2 as a byproduct.
Photosynthesis: Process that converts carbon dioxide into organic compounds using energy from sunlight.
Precambrian: Geologic period extending from ~4.6–0.5 bya covering 7/8th of Earth's history, including the period when life first began.
Proterozoic: Geologic period extending from ~2.5–0.5 bya when the biosphere fluctuated between oxic and anoxic conditions.
Sediment: Materials that are deposited on the bottom of waters (e.g., lakes) typically from settling organisms in the water column and or products of weathering and erosion.
Silurian-Devonian: Geologic period between 0.44–0.40 bya (Silurian) and 0.40–0.35 bya (Devonian). Oxic period when rapid diversification of modern life first began.
Sinkhole: Natural depression or collapse caused by dissolution (erosion) of bedrock such as limestone.
Sulfur cycle: Biogeochemical cycle by which sulfur is exchanged among its different reservoirs on Earth.
References and Recommended Reading
Blankenship, R. et al. The evolutionary transition from anoxygenic to oxygenic photosynthesis. Photosynthesis Research 91, 135-135 (2007).
Cotner, J. B. & Biddanda, B. A. Small players, large role: Microbial influence on biogeochemical processes in pelagic aquatic ecosystems. Ecosystems 5, 105-121 (2002).
Falkowski, P. G. et al. The microbial engines that drive Earth's biogeochemical cycles. Science 230, 1034-1039 (2008).
Grannemann, N. G. et al. The Importance of Ground Water in the Great Lakes Region: Water-Resources Investigations Report 00-4008. United States Geological Survey, 2000.
Grotzinger, J. P. & Knoll, A. H. Stromatolites in Precambrian carbonates: Evolutionary mileposts or environmental dipsticks? Annual Review of Earth and Planetary Sciences 27, 313-358 (1999).
Kump, L. R. The rise of atmospheric oxygen. Nature 451, 277-278 (2008).
Lyons, T. W. et al. Redox redux. Geobiology 7, 489-494 (2009).
Mix, L. et al. "Redox chemistry and metabolic diversity," in The Astrobiology Primer, an Outline of General Knowledge-Version 1. Astrobiology 6, 735-813 (2006).
Nold, S. C. et al. Benthic bacterial diversity in submerged sinkhole ecosystems. Applied and Environmental Microbiology 76, 347-351 (2010).
Ruberg, S. A. et al. Observations of the Middle Island sinkhole in Lake Huron: A unique hydrologic and glacial creation of 400 million years. Marine Technology Society Journal 42, 12-21 (2008).
Sanders, T. G., Jr. et al. Stable isotope analysis reveals benthic macroinvertebrate and fish communities linked to submerged groundwater vents in Lake Huron. Aquatic Biology 12, 1-11 (2011).
Schlesinger, W. H. et al. Introduction to coupled biogeochemical cycles. Frontiers in Ecology and the Environment 9, 5-8 (2011).
Vestal, J. R. Biomass of the cryptoendolithic microbiota from the Antarctic desert. Applied and Environmental Microbiology 54, 947-959 (1988).
Woese, C. R. et al. Towards a natural system of organisms: Proposal for the domains Archaea, Bacteria, and Eucarya. Proceedings of the National Academy of Sciences of the United States of America 87, 4576-4579 (1990).
Whitman, W. B. et al. Prokaryotes: The unseen majority. Proceedings of the National Academy of Sciences of the United States of America 95, 6587-6583 (1998).