« Prev Next »
Introduction
Particle sizes are determined by the mechanism through which the particles enter the atmosphere. For example, homogeneous nucleation, in which a new particle is formed directly from the gas phase, creates particles on the order of a nanometer in diameter (Hirsikko et al., 2011). These particles grow via coagulation or gas-to-particle conversion to hundreds of nanometers. Nucleation and gas-to-particle conversion of material are examples of secondary aerosol formation processes. In contrast, aerosol material emitted to the atmosphere directly is known as primary. Generally, particles with diameters smaller than 100 nm are known as ultrafine or Aitken-mode particles (Seinfeld & Pandis, 2006). Collectively, all particles smaller than 2.5 micrometers (PM2.5) are known as fine aerosols. Particles with diameters between 2.5 and 10 micrometers are correspondingly known as coarse and typically are primary particles generated by physical-mechanical processes (Seinfeld & Pandis, 2006). Aitken-mode particles quickly coagulate and grow due to condensation of vapor species. Because coarse particles settle from the atmosphere relatively rapidly, particles accumulate in the diameter range of hundreds of nanometers and reside in what is known as the accumulation mode (Seinfeld & Pandis, 2006).
Sources of Primary Aerosol
Anthropogenic sources of primary PM include industrial and combustion processes. The nature of industrial particles depends on the process, but combustion particles generally are dominated by black or elemental carbon and heavy organic material such as polycyclic aromatic hydrocarbons (Bond et al. 2007).
Sources of Secondary Aerosol
Gas-to-particle conversion (partitioning to existing PM via adsorption or absorption) is another pathway through which secondary PM is formed (Pankow, 1994). Species such as H2SO4 that have a low vapor pressure can relatively easily undergo such a phase transition. Secondary inorganic PM also can form thermodynamically (salt formation). For example, NH3 can combine with H2SO4, nitric acid (HNO3), or hydrochloric acid to form ammonium salts when the concentrations of these precursor gases are large enough (Tang & Munkelwitz 1994). Because of the hygroscopicity of many inorganic species, water is often taken up, increasing particle size and mass (Petters & Kreidenweis 2007).
Secondary organic aerosol (SOA) is more complex. It is assumed that a mixture of liquid organics provides a medium into which SOA species are absorbed (Pankow 1994). In general, it is believed that the species that undergo this process are functionalized (e.g., carbonyls, carboxylic acids, alcohols, nitrates) oxidation products of primary volatile organic compounds that are present in the atmosphere as a result of both natural and anthropogenic processes (Hallquist et al. 2009). Oligomerization of species in the condensed phase leads to humic-like substances (Kalberer et al. 2004). In addition, certain species heterogeneously react at the surface of a particle and are converted into material that remains in the condensed phase (reactive uptake) (Kroll et al. 2005).
Secondary PM also forms through cloud processing. Clouds continuously undergo evaporation/condensation cycles. During a period in which clouds have significant water content, (partially) soluble species are absorbed from the gas phase into the droplets. Once there, they can be oxidized into species with decreased volatility, such that they remain as particles once the cloud droplets evaporate. This route is believed to be relevant for SOA (Carlton et al. 2006) and secondary sulfate formation, the latter of which is catalyzed by the presence of certain metals in cloud water (Hoffmann & Calvert 1985). An overview of the chemical and physical characteristics, growth and loss mechanisms, and sources of particles in the troposphere is given in Figure 1.
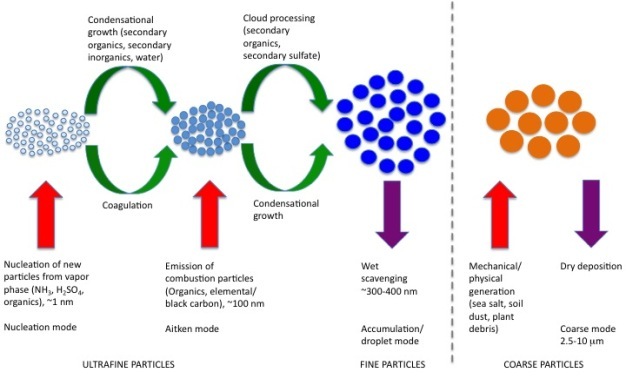

Impacts of PM
Particles cause direct and indirect climate effects. The direct effect on climate results from altering the global radiation balance through the scattering and absorptive properties of particles in the atmosphere (Charlson et al. 1992). PM also influences cloud formation and lifetime. In the upper troposphere, particles serve as ice nuclei (IN) through both homogeneous freezing (direct freezing of a particulate liquid solution) and heterogeneous freezing (freezing caused by the contact of separate liquid and solid phases) mechanisms. Larger, insoluble particles tend to be the most efficient IN, as they provide a lattice upon which ice forms. In the lower troposphere, soluble particles of the appropriate size activate to cloud droplets by behaving as cloud condensation nuclei (CCN) (Twomey 1974; Albrecht 1989; DeMott et al.2010). Activation occurs when particles are exposed to supersaturated water vapor (relative humidity in excess of 100%) and take up enough water to grow to a stable cloud droplet. As the number of CCN increases, the size distribution of the cloud droplets shifts to smaller sizes, increasing cloud reflectivity and lifetime. These cloud-particle connections generally increase Earth's albedo, leading to a change in the planetary radiation balance. Although uncertain, it is believed that this overall aerosol indirect effect (~-0.7 W m-2) combined with forcing associated with the direct effect (~-0.5 W m-2) is on the same order of magnitude as, but opposite in sign, to the climate forcing caused by greenhouse gases (~+1.66 W m-2), as shown in Figure 2 (Intergovernmental Panel on Climate Change, 2007).
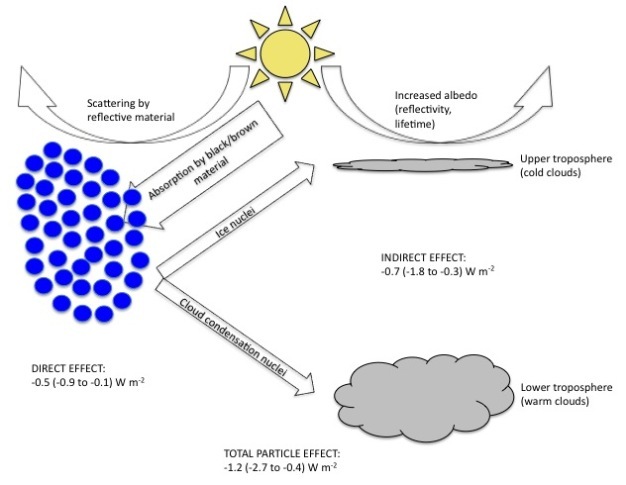

Because of their optical properties, particles significantly impair visibility (White & Roberts, 1977). Accumulation mode particles typically reside in a size domain of approximately 300-400 nm. This corresponds to the wavelength of the visible portion of the solar spectrum. According to Mie theory, particle scattering is enhanced when particle size and light wavelength are similar (Bohren & Huffman 1983). Scattering prevents light from reaching an object in the distance and getting reflected back to a viewer's eye, leading to visibility degradation. Visibility degradation is quantified through an extinction coefficient (bext). In the Beer-Lambert law, bext and a distance determine the decrease in intensity of light of a given wavelength over that distance.
Because of suspension, transport, deposition, and resuspension, particles provide a mechanism by which material is transported between various environmental media and locations. For example, a small part of the nitrogen cycle includes emission of nitric oxide (NO) from soil (Kulmala & Petaja, 2011). The NO is oxidized in the atmosphere to HNO3. As described above, HNO3 can combine with NH3 to form ammonium nitrate salt. This particle deposits to the surface at some location downwind, completing the cycle between the atmosphere and the Earth's surface.
The presence of particles in the atmosphere also affects chemical processes that can occur. For example, in an urban area into which marine air is transported, HNO3 displaces chloride from sea salt, leading to the liberation of chlorine and the formation of sodium nitrate (Gard et al. 1998). The presence of particles provides a surface upon which heterogeneous reactions occur (George & Abbatt 2010). The curvature of particles also enhances photolytic reactions relative to homogeneous photolysis reactions (Nissenson et al. 2006).
The primary motivation behind the establishment by the United States Environmental Protection Agency (USEPA) of National Ambient Air Quality Standards (NAAQS) for both PM2.5 and PM10 (based on mass of particles per volume of air) is the human health consequences suffered by the exposed population. A positive correlation between statistical rates of morbidity and mortality and ambient PM mass concentrations exists (Schwartz et al. 1996).
Inhalation and deposition to the lungs of PM cause or exacerbate cardiovascular issues. Particles that are small enough also cross tissue barriers into the bloodstream and are transported throughout the body. Questions remain regarding the link between human health impacts and specific constituents because of the complex mixture typically associated with PM. It is also possible that the USEPA will move toward a number concentration-based PM NAAQS (Reche et al., 2011). The smallest particles dominate the number concentration of particles but contribute very little to the total particle mass concentration. Therefore, any standard based solely on mass concentration may not protect the population from health impacts of the numerous particles that do not contribute significantly to mass concentration.
Conclusion
References and Recommended Reading
Albrecht, B. A. Aerosols, cloud microphysics and fractional cloudiness. Science 245, 1227-1230 (1989).
Benson, D. R., Yu, J. H. et al. Ternary homogeneous nucleation of H2SO4, NH3, and H2O under conditions relevant to the lower troposphere. Atmospheric Chemistry and Physics 11, 4755-4766 (2011).
Bohren, C. F. & Huffman, D. R. Absorption and Scattering of Light by Small Particles. New York, NY: Wiley, 1983.
Bond, T. C., Bhardwaj, E. et al. Historical emissions of black and organic carbon aerosol from energy-related combustion, 1850-2000. Global Biogeochemical Cycles 21, GB2018 (2007).
Carlton, A. G., Turpin, B. J. et al. Link between isoprene and secondary organic aerosol (SOA): Pyruvic acid oxidation yields low volatility organic acids in clouds. Geophysical Research Letters 33, L06822 (2006).
Charlson, R. J., Schwartz, S. E. et al. Climate forcing by anthropogenic aerosols. Science 255, 423-430 (1992).
DeMott, P. J., Prenni, A. J. et al. Predicting global atmospheric ice nuclei distributions and their impacts on climate. Proceedings of the National Academy of Sciences 107, 11217-11222 (2010).
Despres, V. R., Huffman, J. A. et al. Primary biological aerosol particles in the atmosphere: a review. Tellus Series B - Chemical and Physical Meteorology 64, 15598 (2012).
Gard, E. E., Kleeman, M. J. et al. Direct observation of heterogeneous chemistry in the atmosphere. Science 279, 1184-1187 (1998).
George, I. J. & Abbatt, J. P. D. Heterogeneous oxidation of atmospheric aerosol particles by gas-phase radicals. Nature Geosciences 2, 713-722 (2010).
Hallquist, M., Wegner, J. C. et al. The formation, properties and impact of secondary organic aerosol: current and emerging issues. Atmospheric Chemistry and Physics 9, 5155-5236 (2009).
Hirsikko, A., Nieminen, T. et al. Atmospheric ions and nucleation: a review of observations. Atmospheric Chemistry and Physics 11, 767-798 (2011).
Hoffman, M. R. & Calvert, J. G. Chemical Transformation Modules for Eulerian Acid Deposition Models, Vol. 2, The Aqueous-Phase Chemistry. Research Triangle Park, NC: United States Environmental Protection Agency, 1985.
Intergovernmental Panel on Climate Change. Summary for Policy Makers in Solomon, S., Qin, D. et al. (eds.), Climate Change 2007: The Physical Science Basis. Contribution of Working Group I to the Fourth Assessment Report of the Intergovernmental Panel on Climate Change. New York, NY: Cambridge University Press, 2007.
Kalberer, M., Paulsen, D. et al. Identification of polymers as major components of atmospheric organic aerosols. Science 303, 1659-1662 (2004).
Kirkby, J., Curtius, J. et al. Role of sulphuric acid, ammonia and galactic cosmic rays in atmospheric aerosol nucleation. Nature 476, 429-433 (2011).
Kroll, J. H., Ng, N. L. et al. Chamber studies of secondary organic aerosol growth by reactive uptake of simple carbonyl compounds. Journal of Geophysical Research - Atmospheres 110, D23207 (2005).
Kulmala, M. & Petaja, T. Soil nitrites influence atmospheric chemistry. Nature 333, 1586-1587 (2011).
Lewis, E. R. & Schwartz, S. E. Sea Salt Aerosol Production: Mechanisms, Methods, Measurements, and Models. Washington, DC: American Geophysical Union, 2004.
Mahowald, N. M., Baker, A. R. et al. Atmospheric global dust cycle and iron inputs to the ocean. Global Biogeochemical Cycles 19, GB4025 (2005).
McMurry, P. H., Woo, K. S. et al. Size distributions of 3-10nm atmospheric particles: implications for nucleation mechanisms. Philosophical Transactions of the Royal Society of London Series A - Mathematical Physical and Engineering Sciences 358, 2625-2642 (2000).
Nissenson, P., Knox, C. J. H. et al. Enhanced photolysis in aerosols: evidence for important surface effects. Physical Chemistry Chemical Physics 8, 4700-4710 (2006).
Pandis, S. N., Wexler, A. S. et al. Dynamics of tropospheric aerosols. Journal of Physical Chemistry 99, 9646-9659 (1995).
Pankow, J. F. An absorption model of gas/particle partitioning of organic compounds in the atmosphere. Atmospheric Environment 28, 185-188 (1994).
Petters, M. D. & Kreidenweis, S. M. A single parameter representation of hygroscopic growth and cloud condensation nucleus activity. Atmospheric Chemistry and Physics 7, 1961-1971 (2007).
Reche, C., Querol, X. et al. New considerations for PM, black carbon, and particle number concentration for air quality monitoring across different European cities. Atmospheric Chemistry and Physics 11, 6207-6227 (2011).
Schwartz, J., Dockery D.W. et al. Is daily mortality associated specifically with fine particles? Journal of the Air and Waste Management Association 46, 927-939 (1996).
Seinfeld, J. H. & Pandis, S. N. Atmospheric Chemistry and Physics: From Air Pollution to Climate Change. Hoboken, NJ: John Wiley and Sons, Inc., 2006.
Tang, I. N. & Munkelwitz, H. R. Aerosol phase transformation and growth in the atmosphere. Journal of Applied Meteorology 33, 791-796 (1994).
Twomey, S. Pollution and planetary albedo. Atmospheric Environment 8, 1251-1256 (1987).
Vaattovaara, P., Huttunen, P. E. et al. The composition of nucleation and Aitken mode particles during coastal nucleation events: evidence for marine secondary organic contribution. Atmospheric Chemistry and Physics 6, 4601-4616 (2006).
Whitby, K. T. & Sverdrup, G. M. California aerosols: their physical and chemical characteristics. Advances in Environmental Science and Technology 8, 477-525 (1980).
White, W. H. & Roberts, P. T. On the nature and origins of visibility-reducing aerosols in the Los Angeles basin. Atmospheric Environment 11, 803-812 (1977).
Willeke, K. & Whitby, K. T. Atmospheric aerosols - size distribution interpretation. Journal of the Air Pollution Control Association 25, 529-534 (1975).