Abstract
Population studies in Aotearoa New Zealand found higher bone mineral density and lower rate of hip fracture in people of Polynesian ancestry compared to Europeans. We hypothesised that differences in osteoblast proliferation and differentiation contribute to the differences in bone properties between the two groups. Osteoblasts were cultured from bone samples obtained from 30 people of Polynesian ancestry and 25 Europeans who had joint replacement surgeries for osteoarthritis. The fraction of cells in S-phase was determined by flow cytometry, and gene expression was analysed by microarray and real-time PCR. We found no differences in the fraction of osteoblasts in S-phase between the groups. Global gene expression analysis identified 79 differentially expressed genes (fold change > 2, FDR P < 0.1). Analysis of selected genes by real-time PCR found higher expression of COL1A1 and KRT34 in Polynesians, whereas BGLAP, DKK1, NOV, CDH13, EFHD1 and EFNB2 were higher in Europeans (P ≤ 0.01). Osteoblasts from European donors had higher levels of late differentiation markers and genes encoding proteins that inhibit the Wnt signalling pathway. This variability may contribute to the differences in bone properties between people of Polynesian and European ancestry that had been determined in previous studies.
Similar content being viewed by others
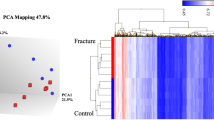
Introduction
Bone health is determined by a combination of factors, including age, genetics, body size and composition, lifestyle, and social determinants1. Ethnicity, a term relating to a person’s cultural affiliation, captures some of these factors, and clinical studies have demonstrated relations between ethnicity and bone health. The most common endpoints of these studies are areal bone mineral density (BMD) and fracture rate, although bone geometry and microarchitecture have also been investigated1.
The current knowledge of ethnic variations in bone properties and health are based on multi-ethnic studies, predominantly from the USA, and several smaller studies of different populations across the world. A large number of studies found that Black women and men have higher BMD and lower fracture rates than their White counterparts2. The largest multi-ethnic study to date is The National Osteoporosis Risk Assessment (NORA) study that determined peripheral BMD and new fractures during the following year in nearly 200,000 women of five different ethnicities3. The study found that Black women had the highest BMD and Asian women had the lowest, whereas White and Hispanic women had the highest risk for fracture. A common finding in studies that compared different ethnic groups is that higher BMD cannot fully account for reduced fracture rates. The NORA study found that after adjusting for multiple covariates, including weight, Asian women had similar BMD to White women, but their fracture risk was considerably lower. Another study found that the absolute incidence of fracture was lower by 30–40% in Black women in comparison to White women with similar BMD4. Bone properties in different ethnic groups have also been studied in Aotearoa New Zealand, a country with a large Polynesian population, comprised of Māori and Pacific peoples. Women of Polynesian ancestry have greater bone mineral content (BMC) and higher BMD than age-matched New Zealand European women, and femoral neck BMD was still about 15% higher in Polynesians after correction for bone size and body mass index5,6. Rates of hip fracture were also substantially lower in Polynesian people7.
Bone structure and material composition are determined by the activity of bone cells through the processes of modelling and remodelling. Three main cell types carry out these processes: osteoblasts that produce the mineralised bone matrix, osteoclasts that resorb bone matrix, and osteocytes that regulate modelling and remodelling of bone. Analyses of gene and protein expression in bone cells have been used to identify molecular mechanisms that underlie bone phenotype. A common source of tissue used in analysis of gene expression in human bone are samples obtained from patients undergoing joint replacement surgeries. As reviewed in Reppe et al.8, most studies compared gene expression between patients who had joint surgeries due to an osteoporotic fracture to those who had surgeries for osteoarthritis. Although differentially expressed genes have been identified, results vary between different studies, and further studies are currently underway8. Ethnic variations in the profile of gene expression in bone have not been investigated.
The current study focused on cellular and molecular mechanisms that could potentially contribute to the observed difference in bone properties between Polynesians and Europeans living in Aotearoa New Zealand. We hypothesised that differences in osteoblast proliferation and gene expression contribute to the differences in bone properties between the groups. We tested this hypothesis using bone samples obtained from patients undergoing total joint replacement surgeries for osteoarthritis.
Results
Study participants
Characteristics of the study participants are presented in Table 1. The mean age of the European group was significantly higher than that of the Polynesian group, whereas the mean body mass index (BMI) was similar in the two groups.
Osteoblasts cultured from the two groups have similar S-phase fraction
In order to test the hypothesis that increased osteoblast proliferation contributes to the favourable bone properties of people of Polynesian ancestry that had been described in the literature6,7, the fraction of cells in different phases of the cell cycle were determined. Osteoblasts cultured from bone fragments were harvested at 50% confluence, labelled with propidium iodide and analysed by flow cytometry. The fractions of cells in S-phase were similar in the Polynesian and European groups (Fig. 1), indicating that when cultured, proliferation rates do not differ substantially between the groups. The fractions of cells in S-phase remained similar between the two groups in further analysis with covariate adjustment for age.
The percentage of cells in S‐phase in osteoblasts cultured from bone fragments. Cells were harvested at 50% confluence, labelled with propidium iodide and analysed by flow cytometry. The ModFit LT software was used to determine the fraction of cells in each phase of the cell cycle. The graph presents individual values, means, and 95% CI. Analysis by t-test found no significant differences between the groups. Figure created in GraphPad Prism 8.2.1, https://www.graphpad.com/.
Global analysis of differential gene expression by microarrays
Global gene expression in osteoblast cultures was determined by GeneChip PrimeView microarrays, using 10 RNA samples from each group. There was no significant age difference between these two groups of 10 participants. The signal distribution on the 20 microarrays and the principal component analysis are presented in supplementary Figures S1 and S2, and a scatter plot of the average level of gene expression from the two groups is presented in supplementary Figure S3. Of the total 79 genes that were differentially expressed (fold change > 2 and FDR-adjusted P value < 0.1), 51 genes had higher expression in the Polynesian group (Table 2) and 28 genes had higher expression in the European group (Table 3). Several of the genes that had the greatest difference in expression levels between the groups had been previously studied in the musculoskeletal system9,10,11. These include HAS1, a gene encoding hyaluronan synthase 1, which was 3.7-fold higher in the Polynesian group, and two genes that had higher expression in the European group: MEGF10, encoding the membrane receptor multiple EGF Like Domains 10, and SEMA6D, encoding semaphorin 6D, which were 5.2- and 3.2-fold higher, respectively.
Further analysis of the microarray results aimed to identify biological processes that are overrepresented in the differentially expressed genes. For this analysis we used a less stringent threshold of unadjusted P < 0.05 instead of the FDR-adjusted P value used above, aiming to include genes that had less stringent differential expression but could be indicative of biological processes of interest when considered as a group. This threshold produced an enriched list of 127 differentially expressed genes (indicated in colour on the scatter plot in Figure S3). Using the PANTHER classification system12, we identified 16 biological processes that were overrepresented in this list (FDR-adjusted P value < 0.05 and fold enrichment > 2.5) (Table 4). Biological processes of potential relevance to bone cell biology included fat cell differentiation (ninefold enrichment, with 5 genes represented on the list of differentially expressed genes), positive regulation of cell adhesion (fourfold enrichment, 11 genes), and regulation of cell migration or motility (threefold enrichment, 17 genes).
Expression analysis of selected genes by real-time PCR
The expression levels of four pre-selected osteoblast marker genes and eight additional genes were determined by real-time PCR in 26 RNA samples from the Polynesian group and 23 samples from the European group. The names of these genes and their differential expression, as determined by the microarray analysis, are presented in Table 5.
Osteoblast marker genes
The expression of four osteoblast marker genes are presented in Fig. 2. COL1A1 (collagen type I, α1 chain) and ALPL (alkaline phosphatase) are expressed in osteoblasts from early stages of differentiation, and BGLAP (osteocalcin), and IBSP (integrin-binding sialoprotein) are markers of advanced differentiation13. COL1A1 had higher expression in the Polynesian group, BGLAP expression was higher in the European group, and ALPL and IBSP had similar expression in the two groups. In post hoc analysis with adjustment for age (ANCOVA), ALPL expression was significantly higher in the Polynesian group (P = 0.037), and all other comparisons remained unchanged.
Comparison of relative expression of osteoblast marker genes between the two groups. Gene expression was determined by real-time PCR using TaqMan assays. The graphs present individual values, medians, and 95% CI. Groups were compared by Mann Whitney test. E, European; P, Polynesian. Figure created in GraphPad Prism 8.2.1, https://www.graphpad.com/.
Other candidate genes
The selection of the eight additional genes was based on their differential expression, as identified by the microarray analysis, and/or their known roles in bone biology. The proteins encoded by DKK1, CDH13, NOV, CCND1, and WISP2 are associated with the canonical Wnt signalling pathway, which plays a central role in the regulation of bone homeostasis. DKK1, CDH13, and NOV, encoding proteins with inhibitory effects on Wnt signalling14,15,16, had higher expression levels in the European group (Fig. 3). CCND1 and WISP2 are target genes of the Wnt pathway17,18, and their expression levels were not significantly different between the groups.
Comparison of relative expression of selected genes between the two groups. Gene expression was determined by real-time PCR using TaqMan assays. The graphs present individual values, medians, and 95%CI. Groups were compared by Mann Whitney test. E, European; P, Polynesian. Figure created in GraphPad Prism 8.2.1, https://www.graphpad.com/.
The remaining three genes tested by real-time PCR were EFNB2, KRT34, and EFHD1. EFNB2, encoding EphrinB2, had higher expression in the European group. EphrinB2 is the main ligand of the Eph4 receptor, which is also expressed in osteoblasts. Interactions between ephrinB2/EphB4 in osteoblasts are required for late stage osteoblast differentiation19. We also determined the expression of KRT34 and EFHD1, two genes that had no documented activity in bone, and were selected for further analysis because of their differential expression on the microarray. Real-time PCR analysis confirmed the differential expression of these genes, with KRT34 showing higher expression in the Polynesian group and EFHD1 showing higher expression in the European group. KRT34 encodes keratin 34, a type I hair keratin. The scientific literature does not provide much information about the role of KRT34 outside hair20. EFHD1 is a calcium-binding protein, localized to the inner mitochondrial membrane21. EFHD1 was shown to modulate apoptosis and differentiation of neuronal and muscle precursor cells22. Although EFHD1 was not studied in osteoblasts, the expression of the related protein EFHD2 was shown to be upregulated during osteoblast differentiation in human mesenchymal cells23.
The results of the differential expression of all eight genes remained similar in post hoc analysis with covariate adjustment for age.
Expression of KRT34 and EFHD1 in differentiating osteoblasts
As a preliminary investigation of the expression of KRT34 and EFHD1 in osteoblasts, we cultured osteoblasts from two additional donors for up to 29 days under conditions that induce differentiation. The expression of KRT34 and EFHD1 was determined at different time points during the differentiation process. KRT34 was highly expressed in undifferentiated osteoblasts, and its expression dropped sharply and stayed low from day 5 onwards (Fig. 4a), whereas EFHD1 expression increased through the differentiation period (Fig. 4b). IBSP expression was also determined in the cells as an indicator of osteoblast differentiation. IBSP expression increased during the culture period, as expected (Fig. 4c).
Expression of KRT34, EFHD1, and IBSP in differentiating osteoblasts. Osteoblasts were cultured for up to 29 days in differentiation medium, and samples were harvested at the indicated time points. Gene expression was determined by real-time PCR. Black line, the level of expression in cells from donor 1; blue line, the level of expression in cells from donor 2. Figure created in GraphPad Prism 8.2.1, https://www.graphpad.com/.
Discussion
This study characterised osteoblasts cultured from bone samples that were obtained from patients undergoing joint replacement surgeries for osteoarthritis, and compared between cells that originated from people who identified as Polynesians and those who identified as Europeans. We found no evidence for difference in cell proliferation rate between the groups. Gene expression analysis by microarray identified 79 differentially expressed genes. Further analysis by real time PCR found that COL1A1 and KRT34 had higher expression in the Polynesian group, while BGLAP, DKK1, NOV, CDH13, EFHD1, and EFNB2 had higher expression in the European group. This differential gene expression may contribute to differences in bone properties that had been determined in these groups in previous studies.
We found that the fraction of cells in S-phase was similar in the two groups, suggesting that the higher BMD in people of Polynesian ancestry does not involve an intrinsic cellular mechanism that accelerates osteoblast proliferation. However, we cannot exclude the possibility that in vivo, differences exist in the proliferative response to extrinsic signals. These potential differences would be missed in our in vitro cultures.
The osteoblast cultures used in this study are primary cultures, and as such are heterogeneous. The method we used for outgrowth cultures from bone fragments is based on the well-established method of growing primary human osteoblastic cells24. In previous studies in our lab, we demonstrated alkaline phosphatase activity in the majority of cells25. In the current study, the high expression of BGLAP, the gene encoding osteocalcin and the most specific osteoblast marker characterised so far, indicated the presence of cells of the osteoblast lineage. The gene expression results suggest that osteoblasts obtained from the two groups vary in their differentiation state under the culture conditions used here. BGLAP and EFNB2, two genes that are upregulated in later stages of osteoblast differentiation13,19, had higher expression in the European group, whereas COL1A1 had higher expression in the Polynesian group. In addition, ALPL, which together with COL1A1 is highly expressed from early differentiation stages, was higher in the Polynesian group after covariate adjustment for age. The expression profile of KRT34 and EFHD1 in osteoblasts have not been described previously, but we found that in long term cultures, under conditions that induced osteoblast differentiation, KRT34 expression declined with time, while EFHD1 expression increased. The higher level of KRT34 and the lower level of EFHD1 in the Polynesian group are consistent with the observation that the osteoblasts from this group demonstrated a less differentiated phenotype than osteoblasts from the European group. It is possible that a prolonged duration of the earlier differentiation stages allows the osteoblasts to produce larger amounts of extracellular matrix. Interestingly, the level of the late marker IBSP13 was similar in the two groups and no additional late markers were identified by the microarray analysis, suggesting that a more subtle tuning of differentiation pathways may exist.
The increased levels of DKK1, CDH13, and NOV in the European group suggest an attenuation of the Wnt signalling pathway, which would negatively affect bone formation14. The proteins encoded by these genes have inhibitory effects on Wnt signalling14,15,16. DKK1 has been studied extensively, as the regulation of the Wnt signalling pathway in bone is considered to be predominantly controlled by DKK1 and the other key Wnt inhibitor, sclerostin (SOST). SOST is only expressed in osteocytes and was undetectable in our osteoblast cultures. CDH13 encodes cadherin 13, that plays a role in cell adhesion and communication, and has been shown to inhibit Wnt signalling by downregulating the expression of the LRP5 receptor16. NOV (CCN3), impairs normal osteoblast differentiation through multiple mechanisms and has been shown to reduce β-Catenin levels and the expression of WNT3 target genes15. Further analysis that focuses on components of the Wnt signalling pathway and its targets could potentially determine the contribution of this pathway to ethnic variation in bone.
Three genes identified by the microarray analysis as having over threefold difference between the groups, had been previously described in the musculoskeletal system, and may contribute to differences in bone properties. HAS1, which had higher expression in the Polynesian group, encodes hyaluronan synthase 1, a key enzyme in the synthesis of the extracellular matrix polysaccharide, hyaluronan. Hyaluronan is synthesised by both osteoblasts and osteoclasts and plays a role in the regulation of bone remodelling, promoting osteoblast activity and suppressing bone resorption26. MEGF10 and SEMA6D had higher expression in the European group. MEGF10 has no known activity in bone, but in muscle it regulates proliferation and migration of myoblasts, via interactions with key components of the Notch signalling pathway9. Recently, variants of the MEGF10 were found to be associated with bone mineral density in children10. SEMA6D, a member of the large family of semaphorins, is a strong inducer of osteoclastogenesis11.
Real time PCR is often used to validate differential gene expression identified by microarray analysis. Here, following the microarray analysis of 10 samples from each group, we determined the expression of 12 genes in 26 samples from the Polynesian group and 23 samples from the European group. In general, the two methods produced congruent results. This excludes BGLAP, that had similar expression levels in the two groups in the microarray analysis, and was significantly higher in the European group in the real-time PCR analysis (P < 0.0001), and WISP2 and CCND1 that were differentially expressed by microarray analysis and were not significantly different between the groups by real-time PCR.
All the molecular investigations in our study focused on RNA, while genomic DNA was not extracted or used in any of our experiments. Studies of genetic variations among human populations have predominantly focused on DNA polymorphisms, providing valuable data, but leaving the functional significance of the variations mostly unexplored. Gene expression profile is closely associated with the distinctive characteristics of a cell, and therefore with its function. Several large-scale gene expression studies that determined differences among human populations used information and samples from the International HapMap Project27,28,29. The relation between genome variation and gene expression suggested that gene expression is heritable, and varies in different human populations. Other studies used a similar approach to that used in our study. For example, a microarray analysis of gene expression in adipose and skeletal muscle tissue samples obtained from European Americans and African Americans, identified 58 differentially expressed genes in muscle and 140 genes in adipose tissue30. To the best of our knowledge, gene expression in bone samples obtained from different human populations has not been examined previously.
By comparing osteoblast properties between Polynesians and Europeans, we aimed to identify molecular mechanisms that contribute to a better bone quality and the lower risk of fracture in the Polynesian population7. Fracture risk is an outcome of multiple factors. Two of these factors, body composition and BMD, have been previously shown to differ between Polynesians and Europeans. People of Polynesian ancestry in Aotearoa New Zealand have a high prevalence of obesity, with greater lean mass and fat mass in comparison to Europeans31,32,33. However, even when groups were weight-matched, Polynesian women had higher BMD at all skeletal sites in comparison to European women5,6. Given the positive relations between weight and BMD, these findings suggest that the overall higher BMD in Polynesian people results from a combination of weight-dependent and weight-independent mechanisms. As the two groups in our study had similar BMI, our result are mostly relevant to potential mechanisms that are independent of weight.
Our study had several limitations. The grouping of the participants by ancestry/ethnicity must be considered carefully. Although ethnic differences in bone density and fracture risk are well documented both globally and in Aotearoa New Zealand, there is little evidence that ethnic categories relate to genetic identity34. In fact, genetic studies consistently find more genetic diversity within ethnic groups than between them34,35. We acknowledge that the ‘self-identified ancestry/ ethnicity’ used in our study has no accurate biomedical meaning and refers predominantly to a social construct34. Another potential limitation of our study is the age difference between the groups analysed for cell fraction in S-phase and gene expression by real time PCR. This limitation was addressed by post hoc sensitivity analysis with covariate adjustment for age, as presented in the “Results” section. Finally, obtaining the bone samples from patients undergoing joint replacement surgeries for OA can be considered as a limitation of the study. Pathological mechanisms in OA affect the whole joint, and characteristic structural changes in bone can be seen in the subchondral region36. Although we avoided subchondral regions when excising the bone samples, we cannot exclude the possibility that the samples were affected by OA. However, OA was not considered a confounding factor in our study, as all the participants had severe OA that required joint replacement surgery.
In summary, our study identified differentially expressed genes in osteoblasts cultured from bone samples obtained from Polynesian and European people. The genes identified here suggest that the duration of stages along the osteoblast differentiation pathway vary between the two groups, and the regulation of the Wnt signalling pathway is altered. These results suggest that there are intrinsic differences between the osteoblast-lineage cell populations obtained from the two groups in this study, but further studies are required to link changes in gene expression, genetics and bone properties.
Methods
Participants
Ethical approval for the study was obtained from the New Zealand Northern A Health and Disability Ethics Committee. All experiments were performed in accordance with the guidelines and regulations of the Committee and all participants provided written informed consent. Participants were undergoing total hip or knee replacement surgeries for osteoarthritis. Exclusion criteria included the use of bone-active medications, metabolic bone disease and fracture of the hip to be replaced. Group allocation was by self-identified ethnicity. The European group included people of European ancestry living in Aotearoa New Zealand. The group of people of Polynesian ancestry living in Aotearoa New Zealand included individuals who identified as Samoan (n = 15), NZ Māori (n = 10), Niuean (n = 2), Cook Island Māori (n = 2) and Tongan (n = 1).
Osteoblast outgrowth cultures
Trabecular bone removed during surgery was cut into small fragments, rinsed several times with PBS and digested with 1 mg/mL collagenase (C6885, Sigma-Aldrich, MO, USA) for 30 min at 37 °C. Bone fragments were then incubated in DMEM/10% FBS (Thermo Fisher Scientific, MA, USA) and 5 μg/mL l-ascorbic acid 2-phosphate (A2P) (Sigma-Aldrich) at 37 °C, 5% CO2 until outgrowth of cells was noted, usually 3–4 days later. Bone fragments were transferred to new flasks and cultured with media replacement every 3–4 days. When the cells were approximately 50% confluent they were harvested for cell cycle analysis and the bone fragments were transferred to new flasks, where the outgrowth of osteoblastic cells continued under the same culture conditions until the cells were 90–100% confluent. Seventy-two hours before the end of the culture period, all bone pieces were removed from the flasks and media renewed.
Analysis of cell cycle by flow cytometry
After harvesting the cells by trypsin digestion, cell pellets were washed once in PBS and then in PBS/1%FCS and resuspended in 200µL of ice cold PBS/1%FBS. Cells were fixed by dropwise addition of 2 mL of ice cold 100% methanol and stored at − 20 °C. Fixed cells were rehydrated by three washes in cold PBS/3%FBS and resuspended in 1 mL PBS/3%FBS. Before analysis, cells were incubated for 10 min with 100 µg/mL RNase and 20 µg/mL propidium iodide (Thermo Fisher Scientific). Analysis of cell DNA content was performed using a BD (Mountain View, CA, USA) FACScan flow cytometer with 10,000 events collected for each analysis. Cell cycle distribution was calculated with the ModFit LT software (Verity Software House, Topsham, ME, USA). Cell cycle was analysed in 21 osteoblast cultures from the Polynesian group and 22 from the European group.
Gene expression profiling
Genomic DNA has not been extracted or investigated in this study. RNA was extracted from cells using the RNeasy Mini-prep Kit with in-column DNase digestion (QIAGEN). RNA quality was determined with RNA StdSens Kit on Experion Automated Electrophoresis System (Bio-Rad). Ten RNA samples from each group, with RNA quality indicator values of 9.7–10, were selected for microarray analysis. Biotinylated, fragmented cRNA was prepared from 210 ng of total RNA using the GeneChip 3′ IVT Express Kit (Affymetrix). cRNA samples were hybridized to GeneChip PrimeView Human Gene Expression Arrays (Affymetrix). Microarray hybridisation, scanning and quality control were carried out at the Auckland Genomics Centre, Faculty of Sciences, University of Auckland.
Real time PCR
Four of the bone samples collected from Polynesian participants and two samples from European participants were excluded from the gene expression analysis because of low RNA quality indicator values, leaving 26 samples from the Polynesian group and 23 from the European group. cDNA was synthesised with SuperScript III Reverse Transcriptase (Thermo Fisher Scientific) from 500 ng of RNA. Gene expression was analysed in multiplex PCR with VIC-labelled TaqMan assay for 18S rRNA as the endogenous control and FAM-labelled TaqMan assay for the target genes (Thermo Fisher Scientific), using the 2−ΔΔCt method.
Osteoblast differentiation
Human osteoblasts grown from bone fragments were seeded in αMEM/10% FBS and 50 µg/mL A2P at a density of 1.5 × 105 cells/well in 6-well plates (Day 0). Once cells reached confluence, about 3 days later, media were changed to αMEM/5% FBS, 50 µg/mL A2P, 10 mM β-glycerophosphate, and 10 nM dexamethasone. Media were changed twice weekly, and cells were maintained for up to 4 weeks. Cells were harvested for RNA extraction at the indicated time points. From day 10 onwards, cells were treated with 120 µg/mL collagenase for 30 min at 37 °C before cell harvest.
Statistical analysis
Transcriptome Analysis Console 4.0.2 (Affymetrix, Inc., Thermo Fisher Scientific, CA, USA) was used for microarray analysis. Differential gene expression was analysed by the Limma Bioconductor package (implemented in Transcriptome Analysis Console 4.0.2), using the Robust Multi-chip Analysis (RMA) algorithm for background adjustment, quantile normalisation, and summarisation. False discovery rate (FDR)-adjusted P values were calculated to control for multiple comparisons. No further adjustments for multiple comparisons were performed. The PANTHER classification system12 was used for classification and enrichment analyses. GraphPad Prism 8.2.1 (GraphPad Software, La Jolla CA, USA) and SAS software (v9.4, SAS Institute Inc, Cary, NC, USA) were used for all other statistical analyses. The statistical tests used are indicated in the figure legends. Post hoc sensitivity analysis was used for the cell cycle and real-time PCR experiments, with adjustment for age (ANCOVA). There was no significant age difference between the two groups analysed by microarrays. Experiments described under cell cycle by flow cytometry and gene expression by real-time PCR were repeated at least twice, and the means of these repeats are presented for each sample in the scatter plots. Tests were 2-tailed with a 5% significance level.
Data availability
Microarray data were submitted to the GEO repository. The records have been assigned the GEO accession number GSE157322.
References
Leslie, W. D. Clinical review: Ethnic differences in bone mass–clinical implications. J. Clin. Endocrinol. Metab. 97, 4329–4340. https://doi.org/10.1210/jc.2012-2863 (2012).
Popp, K. L. et al. Bone mass, microarchitecture and strength are influenced by race/ethnicity in young adult men and women. Bone 103, 200–208. https://doi.org/10.1016/j.bone.2017.07.014 (2017).
Barrett-Connor, E. et al. Osteoporosis and fracture risk in women of different ethnic groups. J. Bone Miner. Res. 20, 185–194. https://doi.org/10.1359/JBMR.041007 (2005).
Cauley, J. A. et al. Bone mineral density and the risk of incident nonspinal fractures in black and white women. JAMA 293, 2102–2108. https://doi.org/10.1001/jama.293.17.2102 (2005).
Cundy, T. et al. Sources of interracial variation in bone mineral density. J. Bone Miner. Res. 10, 368–373. https://doi.org/10.1002/jbmr.5650100306 (1995).
Reid, I. R., Mackie, M. & Ibbertson, H. K. Bone mineral content in Polynesian and white New Zealand women. Br. Med. J. 292, 1547–1548 (1986).
Norton, R. et al. Hip fracture incidence among older people in Auckland: A population-based study. N. Z. Med. J. 108, 426–428 (1995).
Reppe, S., Datta, H. K. & Gautvik, K. M. Omics analysis of human bone to identify genes and molecular networks regulating skeletal remodeling in health and disease. Bone 101, 88–95. https://doi.org/10.1016/j.bone.2017.04.012 (2017).
Saha, M. et al. Consequences of MEGF10 deficiency on myoblast function and Notch1 interactions. Hum. Mol. Genet. 26, 2984–3000. https://doi.org/10.1093/hmg/ddx189 (2017).
Hou, R. et al. Genetic variants affecting bone mineral density and bone mineral content at multiple skeletal sites in Hispanic children. Bone 132, 115175. https://doi.org/10.1016/j.bone.2019.115175 (2020).
Verlinden, L., Vanderschueren, D. & Verstuyf, A. Semaphorin signaling in bone. Mol. Cell Endocrinol. 432, 66–74. https://doi.org/10.1016/j.mce.2015.09.009 (2016).
Mi, H. et al. Protocol Update for large-scale genome and gene function analysis with the PANTHER classification system (v.14.0). Nat. Protoc. 14, 703–721. https://doi.org/10.1038/s41596-019-0128-8 (2019).
Kalajzic, I. et al. Expression profile of osteoblast lineage at defined stages of differentiation. J. Biol. Chem. 280, 24618–24626. https://doi.org/10.1074/jbc.M413834200 (2005).
Baron, R. & Kneissel, M. WNT signaling in bone homeostasis and disease: From human mutations to treatments. Nat. Med. 19, 179–192. https://doi.org/10.1038/nm.3074 (2013).
Ouellet, V. & Siegel, P. M. CCN3 modulates bone turnover and is a novel regulator of skeletal metastasis. J. Cell Commun. Signal 6, 73–85. https://doi.org/10.1007/s12079-012-0161-7 (2012).
Xu, D. et al. Cadherin 13 inhibits pancreatic cancer progression and epithelial-mesenchymal transition by Wnt/beta-catenin signaling. J. Cancer 11, 2101–2112. https://doi.org/10.7150/jca.37762 (2020).
Grünberg, J. R., Hammarstedt, A., Hedjazifar, S. & Smith, U. The novel secreted adipokine WNT1-inducible signaling pathway protein 2 (WISP2) is a mesenchymal cell activator of canonical WNT. J. Biol. Chem. 289, 6899–6907. https://doi.org/10.1074/jbc.M113.511964 (2014).
Iyer, S. et al. FOXOs attenuate bone formation by suppressing Wnt signaling. J. Clin. Investig. 123, 3409–3419. https://doi.org/10.1172/JCI68049 (2013).
Takyar, F. M. et al. EphrinB2/EphB4 inhibition in the osteoblast lineage modifies the anabolic response to parathyroid hormone. J. Bone Miner. Res. 28, 912–925. https://doi.org/10.1002/jbmr.1820 (2013).
Eckhart, L. & Ehrlich, F. In The Hair Fibre: Proteins, Structure and Development (eds Plowman J. E. et al.) 33–45 (Springer Singapore, 2018).
Tominaga, M. et al. Molecular characterization of mitocalcin, a novel mitochondrial Ca2+-binding protein with EF-hand and coiled-coil domains. J. Neurochem. 96, 292–304. https://doi.org/10.1111/j.1471-4159.2005.03554.x (2006).
Dutting, S., Brachs, S. & Mielenz, D. Fraternal twins: Swiprosin-1/EFhd2 and Swiprosin-2/EFhd1, two homologous EF-hand containing calcium binding adaptor proteins with distinct functions. Cell Commun. Signal. 9, 2. https://doi.org/10.1186/1478-811x-9-2 (2011).
Zhang, A. X. et al. Proteomic identification of differently expressed proteins responsible for osteoblast differentiation from human mesenchymal stem cells. Mol. Cell Biochem. 304, 167–179. https://doi.org/10.1007/s11010-007-9497-3 (2007).
Robey, P. G. & Termine, J. D. Human bone cells in vitro. Calcif. Tissue Int. 37, 453–460 (1985).
Naot, D. et al. Differential gene expression in cultured osteoblasts and bone marrow stromal cells from patients with Paget’s disease of bone. J. Bone Miner. Res. 22, 298–309. https://doi.org/10.1359/jbmr.061108 (2007).
Bastow, E. R. et al. Hyaluronan synthesis and degradation in cartilage and bone. Cell Mol. Life Sci. 65, 395–413. https://doi.org/10.1007/s00018-007-7360-z (2008).
The International HapMap Consortium. A haplotype map of the human genome. Nature 437, 1299–1320. https://doi.org/10.1038/nature04226 (2005).
Spielman, R. S. et al. Common genetic variants account for differences in gene expression among ethnic groups. Nat. Genet. 39, 226–231. https://doi.org/10.1038/ng1955 (2007).
Stranger, B. E. et al. Population genomics of human gene expression. Nat. Genet. 39, 1217–1224. https://doi.org/10.1038/ng2142 (2007).
Elbein, S. C. et al. Global gene expression profiles of subcutaneous adipose and muscle from glucose-tolerant, insulin-sensitive, and insulin-resistant individuals matched for BMI. Diabetes 60, 1019–1029. https://doi.org/10.2337/db10-1270 (2011).
Cadzow, M. et al. Lack of direct evidence for natural selection at the candidate thrifty gene locus, PPARGC1A. BMC Med. Genet. 17, 80. https://doi.org/10.1186/s12881-016-0341-z (2016).
Rush, E. C., Freitas, I. & Plank, L. D. Body size, body composition and fat distribution: Comparative analysis of European, Maori, Pacific Island and Asian Indian adults. Br. J. Nutr. 102, 632–641. https://doi.org/10.1017/S0007114508207221 (2009).
Sundborn, G. et al. Overweight and obesity prevalence among adult Pacific peoples and Europeans in the Diabetes Heart and Health Study (DHAHS) 2002–2003, Auckland New Zealand. N. Z. Med. J. 123, 30–42 (2010).
Megyesi, M. S., Hunt, L. M. & Brody, H. A critical review of racial/ethnic variables in osteoporosis and bone density research. Osteoporos. Int. 22, 1669–1679. https://doi.org/10.1007/s00198-010-1503-z (2011).
Kittles, R. A. & Weiss, K. M. Race, ancestry, and genes: Implications for defining disease risk. Ann. Rev. Genomics Hum. Genet. 4, 33–67. https://doi.org/10.1146/annurev.genom.4.070802.110356 (2003).
Loeser, R. F., Goldring, S. R., Scanzello, C. R. & Goldring, M. B. Osteoarthritis: A disease of the joint as an organ. Arthritis Rheum. 64, 1697–1707. https://doi.org/10.1002/art.34453 (2012).
Acknowledgements
This study was funded by the Stevenson Charitable Trust, New Zealand, and by the Wishbone Orthopaedic Research Foundation of New Zealand.
Author information
Authors and Affiliations
Contributions
Conceptualization: J.C., C.M., D.N., R.P.P., I.R.R.; Methodology and Resources: U.B., J.B., K.E.C., A.J.C., R.G., A.H., B.G.M., R.P.P.; Formal analysis and investigation: G.D.G., U.B., A.J.C., B.G.M., D.N.; Writing—original draft preparation: D.N.; Writing—review: J.C., G.D.G., B.G.M., C.M., I.R.R.; Funding acquisition: J.B., D.N., R.P.P., J.C.; All authors reviewed the final version of the manuscript.
Corresponding author
Ethics declarations
Competing interests
The authors declare no competing interests.
Additional information
Publisher's note
Springer Nature remains neutral with regard to jurisdictional claims in published maps and institutional affiliations.
Supplementary Information
Rights and permissions
Open Access This article is licensed under a Creative Commons Attribution 4.0 International License, which permits use, sharing, adaptation, distribution and reproduction in any medium or format, as long as you give appropriate credit to the original author(s) and the source, provide a link to the Creative Commons licence, and indicate if changes were made. The images or other third party material in this article are included in the article's Creative Commons licence, unless indicated otherwise in a credit line to the material. If material is not included in the article's Creative Commons licence and your intended use is not permitted by statutory regulation or exceeds the permitted use, you will need to obtain permission directly from the copyright holder. To view a copy of this licence, visit http://creativecommons.org/licenses/by/4.0/.
About this article
Cite this article
Naot, D., Bentley, J., Macpherson, C. et al. Molecular characterisation of osteoblasts from bone obtained from people of Polynesian and European ancestry undergoing joint replacement surgery. Sci Rep 11, 2428 (2021). https://doi.org/10.1038/s41598-021-81731-5
Received:
Accepted:
Published:
DOI: https://doi.org/10.1038/s41598-021-81731-5
Comments
By submitting a comment you agree to abide by our Terms and Community Guidelines. If you find something abusive or that does not comply with our terms or guidelines please flag it as inappropriate.