Abstract
Organoboron and organosilicon compounds are used not only as synthetic building blocks but also as functional materials and pharmaceuticals, and compounds with multiple boryl and silyl groups are beginning to be used for these purposes. Especially in drug discovery, methodology providing easy stereoselective access to aliphatic nitrogen heterocycles bearing multiple boryl or silyl groups from readily available aromatic nitrogen heterocycles would be attractive. However, such transformations remain challenging, and available reactions have been mostly limited to dearomative hydroboration or hydrosilylation reactions. Here, we report the dearomative triple elementalization (carbo-sila-boration) of quinolines via the addition of organolithium followed by photo-boosted silaboration, affording the desired products with complete chemo-, regio-, and stereoselectivity. The reaction proceeds via the formation of silyl radicals instead of silyl anions. We also present preliminary studies to illustrate the potential of silaboration products as synthetic platforms.
Similar content being viewed by others
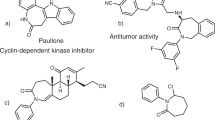
Introduction
Organoboron and organosilicon compounds are attracting attention as optoelectronic materials1,2,3,4 and pharmaceuticals5,6,7,8, in addition to their utility as building blocks in organic synthesis9,10,11,12, and the discovery of cross-coupling reactions for constructing C(sp2)–B and C(sp2)–Si bonds have advanced the use of aryl boron and silicon compounds in these fields13,14. In contrast, although the synthesis of aliphatic boron and silicon compounds is relatively easy with classical methods15,16, their (stereospecific) transformation remains challenging. Nevertheless, C(sp3)–B and C(sp3)–Si bond conversions have begun to be developed, and the products have potential applications in materials science and medicinal chemistry11,12. Furthermore, aliphatic compounds bearing both silyl and boryl groups with different reactivity and properties have potential value as synthetic platforms. Silaboration of alkenes with silylboranes (Si–B) is one of the most straightforward methods for their synthesis17,18,19,20,21. Si–B bond activation is necessary to realize this reaction, though the covalent hetero-interelement Si–B bond is stable and requires high energy to cleave. So far, four Si–B bond activation methods have been developed (Fig. 1a): (i) transition metal-catalyzed activation22,23,24, (ii) base-mediated activation25,26,27,28, (iii) photo-excitation by ultraviolet (UV) light29, and (iv) oxidative activation by a photoredox catalyst30,31. Although the methods involving silyl radical species (iii and iv) are only applicable to hydrosilylation of alkenes, the methods utilizing silyl metal species (i) and silyl anion species (ii) are applicable not only to hydrosilylation but also to silaboration of isolated alkenes.
a Hydrosilylation and silaboration of alkenes using silylborane (Si–B) via four types of Si–B bond activation. b Previous work on dearomative hydroboration, hydrosilylation, or C–Si and N–B bond-forming silaboration of quinolines. c 1,2,3,4-Tetrahydroquinoline natural products and drugs. d Our previous work: quadruple borylation. e This work: dearomative triple elementalization (carbo-sila-boration).
If dearomative C–Si and C–B bond-forming silaboration could be applied to aromatic nitrogen heterocycles instead of simple alkenes, the resulting aliphatic nitrogen heterocycles would be of great interest in the field of medicinal chemistry32,33,34,35,36. However, such transformations are challenging, and available methods have been limited to dearomative hydroboration, hydrosilylation, or C–Si and N–B bond-forming silaboration reactions of aromatic nitrogen heterocycles37,38,39,40,41,42. For example, the construction of the 1,2,3,4-tetrahydroquinoline skeleton, which is a common structure in natural products and pharmaceuticals (Fig. 1c)43,44,45, by reductive hydroboration (Fig. 1b, eqs 1 and 2)40,41, hydrosilylation (Fig. 1b, eq 2)38, or C–Si and N–B bond-forming silaboration (Fig. 1b, eqs 3 and 4)37,42 of quinolines has been reported (Fig. 1b), but the simultaneous introduction of boron and silicon on carbon atoms in this skeleton has not been achieved.
Recently, we developed a UV light-induced B–B bond46 (homo-interelement bond) activation method, achieving quadruple borylation of terminal aryl acetylides (Fig. 1d)47. In this reaction, the B–B bond was excited and smoothly cleaved under UV irradiation by the ate complexation48,49 of diboron (B–B) with light-absorbing aryl acetylene. However, due to the use of highly energetic UV light, the regioselectivity and stereoselectivity cannot be controlled in reactions involving hetero-interelement bonds such as Si–B bonds.
Here, we report the visible-light-induced one-pot dearomative triple elementalization (carbo-sila-boration) of quinolines via (1) the addition of organolithium to quinoline to form lithium anilide I50,51,52,53, (2) ate complexation of I with silylborane II, and (3) direct Si–B bond activation by visible light irradiation to afford the product III (Fig. 1e). This method allows the catalyst-free conversion of readily available quinoline derivatives to 2-alkyl-3-silyl-4-boryl-1,2,3,4-tetrahydroquinoline scaffolds in chemo-, regio-, and stereoselective manner. Experimental and computational studies reveal that this selective reaction proceeds via a Si–B bond activation process, in which visible light excitation of anilide–silylborane ate complex II produces a silyl radical rather than a silyl anion. We also present preliminary studies on the chemo- and stereospecific conversions of C–B/C–Si bonds to C–C, C–O, and C–H/D bonds that serve to illustrate the potential of the silaboration products as synthetic platforms.
Results and discussion
Optimization of reaction conditions
We commenced our study of the dearomative carbo-sila-boration (C/Si/B) by using 6-methoxyquinoline (1a) with a combination of PhMe2Si–B(pin) 2a as a silylborane and nBuLi as an organometal reagent (Table 1). In contrast to our previous reports involving the quadruple borylation of terminal acetylenes47, thermal and Hg lamp irradiation (>250 nm, ultraviolet light) conditions, which would activate the Si–B bond of borate intermediate INT-A generated from 1a, 2a, and nBuLi, resulted in either no reaction or complex mixtures (entries 1–3). These results may be attributed to undesired activation (excitation). To overcome this problem, we envisioned that selective excitation of INT-A by irradiation at an optimal wavelength would facilitate selective Si–B bond cleavage under mild conditions. Our time-dependent density functional theory (TDDFT) calculations of quinoline and model borate complex INT-A’ predicted that the longest absorption band of INT-A’ (366 nm), assigned to the HOMO–LUMO transition, shows a red shift from that of quinoline (289 nm) (Fig. 2). Thus, visible-light irradiation would selectively afford the excited state of ate complex INT-A’* to realize the desired triple elementalization of the quinoline. Pleasingly, the use of a white LED provided the desired carbo-sila-borated (C/Si/B) product (3aa) with 65% yield (entry 4). The structure and stereochemistry of 3aa were unambiguously determined by single-crystal X-ray crystallographic analysis. Notably, the two stereocenters on boron and silane were formed with complete regio- and diastereoselectivity (>99:1), as confirmed by 1H-NMR analysis of the crude product.
We surveyed various types of light sources to facilitate the Si–B bond cleavage process and found that the yield of 3aa was drastically improved to 80% when blue LEDs were used (entry 5). Importantly, the yield significantly decreased under dark conditions (entry 6). Examination of several solvents revealed dioxane to be much superior to THF, hexane, or toluene (entries 7–9). Increasing the amount of silylborane 2a (3 equiv) promoted the reaction to afford the desired product in the highest yield of 88% (entry 10). These conditions were found to be optimal.
Dearomative triple elementalization of quinolines
With the optimized conditions in hand, we next investigated the substrate scope of this triple elementalization (Fig. 3a). A variety of quinolines could be employed: (1) the position (6-, 7-, 8-, or 9-) of the methyl group on the benzene part had little impact on the reaction (3ca, 3da, 3ea, and 3fa); (2) quinolines with electron-donating substituents (OMe, alkyl, amino, and silyl groups; 3aa, 3ca, 3ia, 3ka, and 3la) as well as electron-withdrawing substituents (fluoro, chloro, phenyl, and thienyl groups; 3ga, 3ja, 3ma, and 3na) at the 6-position of the benzene part were efficiently converted to the corresponding carbo-sila-borated products in moderate to excellent yields; (3) π-extended aromatics 1o and 1p were also available; (4) 4- and 3-substituents (1q and 1r) on the pyridine part were tolerated, affording 1,2,3,4-tetrahydroquinolines with tetrasubstituted stereocenters, though 2-methylquinoline was unreactive. The stereochemistry of the tertiary boronic ester 3qa was unambiguously determined by single-crystal X-ray crystallographic analysis. For the silyl substituents, not only the dialkylarylsilyl group but also trialkylsilyl (3ab and 3ac) and hydrosilyl (3ad) groups were compatible. In addition, various alkylations, including n-butylation (3aa), methylation (3ae), phenylation (3af), and sec-butylation (3ag) could be employed in the first dearomative alkylation step to afford the corresponding products. The starting quinolines 1 were not recovered in the reactions shown in Fig. 3a. Some N-functionalizations of 1,2,3,4-tetrahydroquinolines, such as the acetyl (3aa) and the p-methyl or p-nitrobenzoyl (3ah and 3ai) substituted compounds, were available in one pot without further purification. However, the non-substituted product (3aj) obtained without acyl chloride and the N-methylated product (3ak) synthesized using iodomethane instead of acyl chloride were only detected as major products by 1H-NMR and ESI-MS of the crude mixtures and could not be isolated due to their instability. Importantly, all the products listed in Fig. 3a were obtained with complete regio- and diastereoselectivity.
a Synthesis of carbo-sila-borated tetrahydroquinolines. b Synthesis of carbo-diborated tetrahydroquinoline. Reactions were performed on a 0.25 mmol (1) scale under argon. N–Functionalization using R3Cl was performed at 50–80 °C until the reaction was completed. The yields were determined by 1H-NMR analysis. aRun for 48 h. bRun for 96 h. cTHF was used instead of dioxane as a solvent. d370 nm LEDs were used instead of blue LEDs as a light source. eIodomethane was used instead of acyl chloride at 80 °C.
We also investigated a dearomative carbo-diboration reaction (Fig. 3b). The use of bis(pinacolate)diboron (B–B) 4 instead of silylborane 2 gave the diborate intermediate INT-F. Blue LED irradiation of INT-F led to a carbo-diboration reaction, giving carbo-diboration product 5a with good diastereoselectivity (85:15) as confirmed by 1H NMR analysis of the crude reaction mixture (Fig. 3b). The stereochemistry of the major diastereomer of 5a was confirmed by X-ray crystallography. These reactions were completely shut down in the dark, indicating that the present selective photoexcitation strategy is also essential for the B–B bond activation.
Silaborated tetrahydroquinolines as synthetic platforms
We have also obtained a variety of highly functionalized 1,2,3,4-tetrahydroquinoline derivatives by preparative-scale synthesis and chemo- and stereospecific transformations of 2-alkyl-3-silyl-4-boryl-1,2,3,4-tetrahydroquinoline 3aa (Fig. 4a). The dearomative triple elementalization of 6-methoxyquinoline (1a, 152 mg, 1 mmol) using an additional electrophile [(pin)BOiPr] smoothly proceeded to give the desired 1,2,3,4-tetrahydroquinoline 3aa in 72% yield. The pinacol group on the boryl moiety of 3aa could be easily removed by KF to give trifluoroborate salt 6 in good yield54. For selective transformation of the boryl group, hydrodeboration or deuterodeboration proceeded under basic conditions using NaOMe to afford the corresponding products 7-H (C/Si/H) and 7-D (C/Si/D). The direct oxidation of 3aa afforded alcohol 8 (C/Si/O), which was further converted to methyl ether 9 and benzyl ether 10. Alkylation using MeI and NaOMe provided methylation product 11 (C/Si/C) with retention of the stereochemistry. Transformation of the silyl group was also achieved: the highly sterically hindered C–Si bond of 11 was smoothly oxidized to a C–O bond via fluorosilane intermediate 12 under the standard conditions of Tamao–Fleming oxidation to afford the corresponding alcohol 13 (C/O/C). Furthermore, the present photo-boosted dearomative triple elementalization and subsequent derivatization could be combined with the asymmetric alkylation of quinolines reported by the Alexakis group55, affording enantioenriched 2-alkyl-3-silyl-4-boryl-1,2,3,4-tetrahydroquinoline (2S,3R,4S)-(+)-3aa and 1,2,3,4-tetrahydroquinoline-4-ol (2S,3S,4S)-(+)-8 with excellent enantioselectivity (up to 99% ee, Fig. 4b). These preparative-scale reactions and product transformations suggest that silaborated 1,2,3,4-tetrahydroquinoline derivatives have considerable potential as synthetic platforms.
Experimental and theoretical mechanistic studies
Next, we sought to acquire mechanistic insights into the triple elementalization reaction. Firstly, control experiments were performed as shown in Fig. 5a. The reaction was inhibited by a single-electron-transfer (SET) inhibitor [nitrobenzene (14)] and by radical scavengers [1,2-diphenylethylene (15) and (2,2,6,6-tetramethylpiperidin-1-yl)oxy (TEMPO, 16)], but not by an excited triplet-state quencher 17. These results indicate that this reaction involves both excited-state species and free radical species. We also identified the adducts of the in-situ-generated silyl radical56 with TEMPO 1657 and with aliphatic alkene (tert-butyl 4-methylenepiperidine-1-carboxylate, S1) by means of GCMS or ESI-MS measurements, as shown in Supplementary Figs. 5, 6. Competition experiments using two types of combinations of silylboranes (2a and 2b) and quinolines (1a and 1g) resulted in no detection of the cross-reaction products (3gb and 3aa), which suggests that the borate intermediate undergoes intramolecular reaction (Fig. 5b). In addition, as depicted in Fig. 5c, the addition of 9,10-dihydroanthracene as a hydrogen source before the light irradiation afforded not only the desired product (3aa) but also 2-alkyl-3-silyl-1,2,3,4-tetrahydroquinoline 7-H as the hydrogenated intermediate in 25% yield. This result indicates that the present triple elementalization consists of the following three events; (1) dearomative alkylation, (2) photo-induced intramolecular silylmetalation of 1,2-dihydroquinoline, and (3) intramolecular borylation of the benzyl anion species.
Based on the experimental studies, a plausible mechanism is illustrated in Fig. 5d. As already mentioned, nucleophilic silylation from the borate intermediate INT-A did not proceed effectively. Instead, INT-A could be selectively excited by photo-irradiation to a singlet excited state (S1; 1INT-A*), which enables cleavage (homolysis) of the Si–B bond to proceed smoothly, generating both silyl radical and radical anion species 3INT-B. Indeed, TD-DFT calculation of INT-A indicates that the charge-transfer character from the HOMO composed of the σ orbital of the Si–B bond and the π orbital of the 1,2-dihydroquinoline moiety to the π* orbital of the 1,2-dihydroquinoline moiety (LUMO) is predominant in the visible absorption range. Then, radical coupling yields the corresponding silylmetalated intermediate INT-C. Finally, the boryl group transfer from the nitrogen atom to the carbon atom via bicyclo intermediate INT-D affords the desired triple elementalization (C/Si/B) product INT-E. The diastereoselectivity of the boryl and silyl groups would be determined by steric hindrance in INT-D’, resulting in the anti-configuration of these groups.
To gain detailed insight into the proposed mechanisms, model calculations using borate complex IM1, which is generated from 1b, 2a, and ethyllithium (EtLi) coordinated with two dimethyl ethers (model solvent), were performed as shown in Fig. 6. Borate complex IM1 facilitates cleavage of the Si–B bond to generate silyllithium (silyl anion) species IM2, which is stabilized by the π orbital of the styrene unit and the lone pair of the nitrogen atom. Then, the addition of this silyllithium species to the styrene moiety affords the corresponding silylmetalated intermediate IM3. However, these events both suffer from high activation energies (+23.0 and +29.8 kcal mol–1, respectively), mainly due to the stability of the Si–B bond (TS1_2) and the steric bulkiness of the quinoline skeleton and the silyllithium species (TS1_2 and TS2_3). In contrast, our TDDFT calculation of IM1 in the excited S1 state indicates that a large energy gain would facilitate smooth cleavage of the Si–B bond to form 3IM4* via 3IM1* with a small activation energy of 2.5 kcal mol–1. The resulting silyl atom shows the nature of a “silyl radical.” The subsequent silyl radical addition to the styrene moiety (3IM4* → 3IM5*) requires a smaller activation energy (16.3 kcal mol−1) than the corresponding silyl anion addition (IM2 → IM3), resulting in the silylmetalated intermediate 3IM5*. The structure of 3TS4_5* clearly shows that the steric interaction between the quinoline skeleton and the silyl substituent is smaller than that of TS2_3. These steps complete the C(sp3)–Si bond formation, leading to silylmetalated intermediate IM6, which is in equilibrium with the intermediate IM3.
a Energy diagram using Gibbs free energies. b Chemical structures of intermediates and transition states. Gibbs free energy changes are shown in kcal mol–1. DFT Calculations were performed at the (U)B3LYP/6-31+G*&PCM(1,4-dioxane)//(U)B3LYP/6-31+G* level of theory. [Li] = Li(OMe2)2. IM intermediate, TS transition state.
In addition, a reaction pathway for C(sp3)–B bond formation was also identified by DFT calculations. The transfer of the boryl group from the nitrogen atom to the carbon atom (IM6 → IM8) involves two transition states (TS6_7 and TS7_8) for C(sp3)–B bond formation and N–B bond cleavage, respectively, both of which are kinetically and thermodynamically favorable (∆G‡ < 20 kcal/mol and ∆G < 0 kcal/mol) in the ground state. On the other hand, the C(sp3)–B bond formation in the triplet state (3IM5* → 3IM9* → 3IM10*) is kinetically and thermodynamically unfavored. These calculations are in good agreement with the experimental results, and thus we can conclude that in situ generations of radical species from the excited borate complex facilitates the present hetero-elementalization reaction.
Dearomative triple elementalization of anthracene and phenanthrene
Finally, based on the mechanisms proposed in Fig. 5d, we investigated another type of dearomative triple elementalization. The use of 9-bromoanthracene (18) instead of quinoline (1) yielded borate intermediate INT-G via a halogen–lithium exchange reaction using nBuLi (Fig. 7a). As in the case of quinoline, TDDFT calculations for anthracene and the model borate complex INT-G’ predicted that the longest and the second longest absorption bands of INT-G’ (532 and 396 nm) are due to the HOMO–LUMO and HOMO-1–LUMO transitions, respectively, showing redshifts from anthracene (385 nm) (Fig. 7b). Both the transitions show charge-transfer character from the HOMO or the HOMO-1, consisting of the σ orbital of the Si–B bond and the π orbital of the anthracene moiety, to the π* orbital (LUMO) of the anthracene moiety. Gratifyingly, irradiation of INT-G with blue LEDs followed by alkylation of INT-H with n-butyl bromide led to the carbo-sila-boration reaction, affording 9-silyl-10-alkyl-boryl-9,10-dihydroanthracene 19 in 60% yield with complete regio- and stereoselectivity. The diastereoselectivity is expected to be determined by the electrophilic addition of n-butyl bromide at the sterically unhindered side, resulting in the anti-configuration of the n-butyl and silyl groups. Using bis(pinacolato)diboron (4, B–B) instead of silylborane 2a, carbo-diboration of anthracene via borate complex INT-I afforded 9-boryl-10-alkyl-boryl-9,10-dihydroanthracene 20 in 75% yield and a diastereomeric ratio of 60:40 as confirmed by 1H NMR analysis of the crude product (Fig. 7c). Dearomative carbo-diboration of 9-bromophenanthrene (21) instead of 18 also proceeded to give 9-boryl-10-alkyl-boryl-9,10-dihydrophenanthrene 22 in 72% yield via borate complex INT-J (Fig. 7d). However, dearomative carbo-diboration and carbo-sila-boration of 1-bromonaphthalene did not proceed, probably due to the high aromaticity of the naphthalene ring. Thus, the present Si–B/B–B bond photoactivation strategy may be useful for the functionalization of various polycyclic aromatic hydrocarbons.
a Synthesis of carbo-sila-borated dihydroanthracene. b Molecular orbital and UV/vis absorption spectrum of INT-G’ by (TD)DFT calculations at the B3LYP/6-31+G* level of theory. c Synthesis of carbo-diborated dihydroanthracene. d Synthesis of carbo-sila-borated dihydrophenanthrene. Reactions were performed on a 0.25 mmol (18 or 21) scale under argon. Yields were determined by 1H-NMR analysis.
In conclusion, we have accomplished chemo-, regio-, and stereoselective dearomative triple elementalization (carbo-sila-boration) of quinolines by the addition of organolithium followed by photo-boosted silaboration. Experimental and computational studies indicate that the selective photoexcitation of borate complexes results in smooth cleavage of the Si–B bond to form silyl radicals rather than silyl anions, enabling carbo-sila-boration (C/Si/B) to occur. Although chemo- and stereoselective transformations of adjacent boron–silicon functional groups are still under study, carbo-sila-borated tetrahydroquinolines are expected to be versatile synthetic platforms; for example, the conversion of boryl and silyl groups yields carbo-oxy-silylation (C/Si/O), carbo-sila-deuteration (C/Si/D), dicarbo-silylation (C/Si/C), and dicarbo-oxidation (C/O/C) products in a regio- and stereoselective manner.
Methods
General procedure for dearomative carbo-sila-boration of quinolones
Quinoline 1 (0.25 mmol) was charged in a dried Schlenk tube and dissolved in dry dioxane (0.50 mL). To the mixture was added organolithium (0.25 mmol; 2.55 M in hexane solution) at 0 °C. The solution was stirred for 2 h, and then silylborane 2 (0.75 mmol) was added at room temperature. The reaction tube was sealed and irradiated with blue LEDs equipped with a cooling fan at room temperature for 24 hours. N-Acylation reagent (1.0–1.5 mmol) was then added to the mixture at room temperature and the resulting mixture was stirred at 50 °C for 24 h. The reaction was quenched with water (2 mL) and the mixture was extracted with ethyl acetate (10 mL × 3). The ethyl acetate layers were combined and dried over MgSO4, and the solvent was removed under reduced pressure. The residue was purified by preparative thin-layer chromatography.
Data availability
The crystallographic data generated in this study have been deposited in the Cambridge Crystallographic Data Centre under accession code CCDC 2184597 (3aa), CCDC 2184600 (3qa), and CCDC 2184598 (5a). Cartesian coordinates of intermediates and transition states are available in Supplementary Data 1. All of the other data supporting the findings of this study are provided in the main text or the Supplementary Information.
References
Hatakeyama, T. et al. Ultrapure blue thermally activated delayed fluorescence molecules: efficient HOMO–LUMO separation by the multiple resonance effect. Adv. Mater. 28, 2777–2781 (2016).
Saito, S., Matsuo, K. & Yamaguchi, S. Polycyclic π-electron system with boron at its center. J. Am. Chem. Soc. 134, 9130–9133 (2012).
Zhao, Z., Hea, B. & Tang, B. Z. Aggregation-induced emission of siloles. Chem. Sci. 6, 5347–5365 (2015).
Marro, E. A. & Klausen, R. S. Conjugated polymers inspired by crystalline silicon. Chem. Mater. 31, 2202–2211 (2019).
Smoum, R., Rubinstein, A., Dembitsky, V. M. & Srebnik, M. Boron containing compounds as protease inhibitors. Chem. Rev. 112, 4156–4220 (2012).
Fernandes, G. F. S., Denny, W. A. & Dos Santos, J. L. Boron in drug design: recent advances in the development of new therapeutic agents. Eur. J. Med. Chem. 179, 791–804 (2019).
Franz, A. K. & Wilson, S. O. Organosilicon molecules with medicinal applications. J. Med. Chem. 56, 388–405 (2013).
Ramesh, R. & Reddy, D. S. Quest for novel chemical entities through incorporation of silicon in drug scaffolds. J. Med. Chem. 61, 3779–3798 (2018).
Miyaura, N. & Suzuki, A. Palladium-catalyzed cross-coupling reactions of organoboron compounds. Chem. Rev. 95, 2457–2483 (1995).
Denmark, S. E. & Sweis, R. F. Design and implementation of new, silicon-based, cross-coupling reactions: importance of silicon–oxygen bonds. Acc. Chem. Res. 35, 835–846 (2002).
Sandford, C. & Aggarwal, V. K. Stereospecific functionalizations and transformations of secondary and tertiary boronic esters. Chem. Commun. 53, 5481–5494 (2017).
Komiyama, T., Minami, Y. & Hiyama, T. Recent advances in transition-metal-catalyzed synthetic transformations of organosilicon reagents. ACS Catal. 7, 631–651 (2017).
Mkhalid, I. A. I., Barnard, J. H., Marder, T. B., Murphy, J. M. & Hartwig, J. F. C–H activation for the construction of C–B bonds. Chem. Rev. 110, 890–931 (2010).
Cheng, C. & Hartwig, J. F. Catalytic silylation of unactivated C–H bonds. Chem. Rev. 115, 8946–8975 (2015).
Obligacion, J. V. & Chirik, P. J. Earth-abundant transition metal catalysts for alkene hydrosilylation and hydroboration. Nat. Rev. Chem. 2, 15–34 (2018).
Hofmann, R. J., Vlatkovic, M. & Wiesbrock, F. Fifty years of hydrosilylation in polymer science: a review of current trends of low-cost transition-metal and metal-free catalysts, non-thermally triggered hydrosilylation reactions, and industrial applications. Polymers 9, 534–570 (2017).
Suginome, M. & Ito, Y. Transition-metal-catalyzed additions of silicon–silicon and silicon–heteroatom bonds to unsaturated organic molecules. Chem. Rev. 100, 3221–3256 (2000).
Oestreich, M., Hartmann, E. & Mewald, M. Activation of the Si–B interelement bond: mechanism, catalysis, and synthesis. Chem. Rev. 113, 402–441 (2013).
Cuenca, A. B., Shishido, R., Ito, H. & Fernández, E. Transition-metal-free B–B and B–interelement reactions with organic molecules. Chem. Soc. Rev. 46, 415–430 (2017).
Ansell, M. B., Navarro, O. & Spencer, J. Transition metal catalyzed element–element additions to alkynes. Coord. Chem. Rev. 336, 54–77 (2017).
Feng, J.-J., Mao, W., Zhang, L. & Oestreich, M. Activation of the Si–B interelement bond related to catalysis. Chem. Soc. Rev. 50, 2010–2073 (2021).
Suginome, M., Nakamura, H. & Ito, Y. Platinum-catalyzed regioselective silaboration of alkenes. Angew. Chem. Int. Ed. Engl. 36, 2516–2518 (1997).
Ohmura, T., Furukawa, H. & Suginome, M. Ligand-controlled, complementary stereoselectivity in the platinum-catalyzed intramolecular silaboration of alkenes. J. Am. Chem. Soc. 128, 13366–13367 (2006).
Kamei, T., Nishino, S. & Shimada, T. Ni-catalyzed hydroboration and hydrosilylation of olefins with diboron and silylborane. Tetrahedron Lett. 59, 2896–2899 (2018).
O’Brien, J. M. & Hoveyda, A. H. Metal-free catalytic C–Si bond formation in an aqueous medium. Enantioselective NHC-catalyzed silyl conjugate additions to cyclic and acyclic α,β-unsaturated carbonyls. J. Am. Chem. Soc. 133, 7712 (2011).
Ito, H., Horita, Y. & Yamamoto, E. Potassium tert-butoxide-mediated regioselective silaboration of aromatic alkenes. Chem. Commun. 48, 8006–8008 (2012).
Wu, H. et al. Mechanism of NHC-catalyzed conjugate additions of diboron and borosilane reagents to α,β-unsaturated carbonyl compounds. J. Am. Chem. Soc. 137, 10585–10602 (2015).
Morimasa, Y., Kabasawa, K., Ohmura, T. & Suginome, M. Pyridine-based organocatalysts for regioselective syn-1,2-silaboration of terminal alkynes and allenes. Asian J. Org. Chem. 8, 1092–1096 (2019).
Matsumoto, A. & Ito, Y. New generation of organosilyl radicals by photochemically induced homolytic cleavage of silicon–boron bonds. J. Org. Chem. 65, 5707–5711 (2000).
Zhong, M., Pannecoucke, X., Jubault, P. & Poisson, T. Copper-photocatalyzed hydrosilylation of alkynes and alkenes under continuous flow. Chem. Eur. J. 27, 11818–11822 (2021).
Takemura, N., Sumida, Y. & Ohmiya, H. Organic photoredox-catalyzed silyl radical generation from silylboronate. ACS Catal. 12, 7804–7810 (2022).
Wertjes, W. C., Southgate, E. H. & Sarlah, D. Recent advances in chemical dearomatization of nonactivated arenes. Chem. Soc. Rev. 47, 7996–8017 (2018).
Liu, Y.-Z., Song, H., Zheng, C. & You, S.-L. Cascade asymmetric dearomative cyclization reactions via transition-metal-catalysis. Nat. Synth. 1, 203–216 (2022).
Cheng, Y.-Z., Feng, Z., Zhang, X. & You, S.-L. Visible-light induced dearomatization reactions. Chem. Soc. Rev. 51, 2145–2170 (2022).
Tan, G. et al. Photochemical single-step synthesis of β-amino acid derivatives from alkenes and (hetero)arenes. Nat. Chem. 14, 1174–1184 (2022).
Schlosser, M. & Mongin, F. Pyridine elaboration through organometallic intermediates: regiochemical control and completeness. Chem. Soc. Rev. 36, 1161–1172 (2007).
Oshima, K., Ohmura, T. & Suginome, M. Palladium-catalyzed regioselective silaboration of pyridines leading to the synthesis of silylated dihydropyridines. J. Am. Chem. Soc. 133, 7324–7327 (2011).
Gandhamsetty, N., Joung, S., Park, S.-W., Park, S. & Chang, S. Boron-catalyzed silylative reduction of quinolines: selective sp3 C–Si bond formation. J. Am. Chem. Soc. 136, 16780–16783 (2014).
Kubota, K., Watanabe, Y., Hayama, K. & Ito, H. Enantioselective synthesis of chiral piperidines via the stepwise dearomatization/borylation of pyridines. J. Am. Chem. Soc. 138, 4338–4341 (2016).
Kubota, K., Watanabe, Y. & Ito, H. Synthesis of enantiomerically enriched chiral tetrahydroquinolines via sequential dearomatization/enantioselective borylation reactions. Adv. Synth. Catal. 358, 2379–2384 (2016).
Kim, E., Jeon, H. J., Park, S. & Chang, S. Double hydroboration of quinolines via borane catalysis: diastereoselective one pot synthesis of 3-hydroxytetrahydroquinolines. Adv. Synth. Catal. 362, 308–313 (2020).
Jeong, E., Heo, J., Jin, S., Kim, D. & Chang, S. KOtBu-Catalyzed 1,2-silaboration of N–heteroarenes to access 2-silylheterocycles: a cooperative model for the regioselectivity. ACS Catal. 12, 4898–4905 (2022).
O’Hagan, D. Pyrrole, pyrrolidine, pyridine, piperidine and tropane alkaloids. Nat. Prod. Rep. 17, 435–446 (2000).
Sridharan, V., Suryavanshi, P. A. & Meneńdez, J. C. Advances in the chemistry of tetrahydroquinolines. Chem. Rev. 111, 7157–7259 (2011).
Muthukrishnan, I., Sridharan, V. & Meneńdez, J. C. Progress in the chemistry of tetrahydroquinolines. Chem. Rev. 119, 5057–5191 (2019).
Cid, J., Gulyás, H., Carbó, J. J. & Fernández, E. Trivalent boron nucleophile as a new tool in organic synthesis: reactivity and asymmetric induction. Chem. Soc. Rev. 41, 3558–3570 (2012).
Yukimori, D., Nagashima, Y., Wang, C., Muranaka, A. & Uchiyama, M. Quadruple borylation of terminal alkynes. J. Am. Chem. Soc. 141, 9819–9822 (2019).
Tilly, D., Chevallier, F., Mongin, F. & Gros, P. C. Bimetallic combinations for dehalogenative metalation involving organic compounds. Chem. Rev. 114, 1207–1257 (2014).
Uzelac, M. & Hevia, E. Polar organometallic strategies for regioselective C–H metallation of N-heterocyclic carbenes. Chem. Commun. 54, 2455–2462 (2018).
Boudier, A., Bromm, L. O., Lotz, M. & Knochel, P. New applications of polyfunctional organometallics in organic synthesis. Angew. Chem. Int. Ed. 39, 4414–4435 (2000).
Ila, H., Baron, O., Wagner, A. J. & Knochel, P. Functionalized magnesium organometallics as versatile intermediates for the synthesis of polyfunctional heterocycles. Chem. Commun. 52, 583–593 (2006).
Chen, Q., du Jourdin, M. & Knochel, P. Transition-metal-free BF3-mediated regioselective direct alkylation and arylation of functionalized pyridines using Grignard or organozinc reagents. J. Am. Chem. Soc. 135, 4958–4961 (2013).
Chen, Q., Leoń, T. & Knochel, P. Transition-metal-free BF3-mediated oxidative and non-oxidative cross-coupling of pyridines. Angew. Chem. Int. Ed. 53, 8746–8750 (2014).
Lennox, A. J. J. & Lloyd-Jones, G. C. Preparation of organotri-fluoroborate salts: precipitation-driven equilibrium under non-etching conditions. Angew. Chem. Int. Ed. 51, 9385–9388 (2012).
Amiot, F., Cointeaux, L., Silve, E. J. & Alexakis, A. Enantioselective nucleophilic addition of organometallic reagents to quinoline: regio-, stereo- and enantioselectivity. Tetrahedron 60, 8221–8231 (2004).
Li, J.-S. & Wu, J. Recent developments in the photo-mediated generation of silyl radicals and their application in organic synthesis. ChemPhotoChem 2, 839–846 (2018).
Lv, H. et al. Photocatalyzed cross-dehydrogenative coupling of silanes with alcohols and water. Chem. Commun. 57, 3660–3663 (2021).
Acknowledgements
The authors are grateful for Grants-in-Aid for Scientific Research (Nos. JP20K22521, JP21K14623, and JP22H05346 to Y.N., Nos. JP22H00320, JP22H05125, and JP17H06173 to M.U., and No. JP19H00893 to K.T.) from JSPS (Japan), as well as grants from JST CREST (No. JPMJCR19R2), Japan Agency for Medical Research and Development (AMED) (No. JP22nk0101111), NAGASE Science Technology Foundation, Naito Foundation, Chugai Foundation, (to M.U.), Fukuoka Naohiko Memorial Foundation, UBE Foundation (to Y.N.), and Uehara Memorial Foundation (to Y.N. and M.U.). A generous allotment of computational resources from TSUBAME (Tokyo Institute of Technology) is gratefully acknowledged.
Author information
Authors and Affiliations
Contributions
Y.N. conceived and designed the experiments. S.I., Y.N., and D.Y. conducted the experiments. J.T. assisted with the experiments. S.I., Y.N., and M.U. conducted computational studies. Y.N., T.M., and K.M. performed the X-ray crystal structure analysis. Y.N., M.U., and K.T. directed the project and wrote the manuscript. All authors participated in data analyses and discussions.
Corresponding authors
Ethics declarations
Competing interests
The authors declare no competing interests.
Peer review
Peer review information
Nature Communications thanks Jin Kyoon Park and the other, anonymous, reviewer(s) for their contribution to the peer review of this work.
Additional information
Publisher’s note Springer Nature remains neutral with regard to jurisdictional claims in published maps and institutional affiliations.
Supplementary information
Rights and permissions
Open Access This article is licensed under a Creative Commons Attribution 4.0 International License, which permits use, sharing, adaptation, distribution and reproduction in any medium or format, as long as you give appropriate credit to the original author(s) and the source, provide a link to the Creative Commons license, and indicate if changes were made. The images or other third party material in this article are included in the article’s Creative Commons license, unless indicated otherwise in a credit line to the material. If material is not included in the article’s Creative Commons license and your intended use is not permitted by statutory regulation or exceeds the permitted use, you will need to obtain permission directly from the copyright holder. To view a copy of this license, visit http://creativecommons.org/licenses/by/4.0/.
About this article
Cite this article
Ishigaki, S., Nagashima, Y., Yukimori, D. et al. Dearomative triple elementalization of quinolines driven by visible light. Nat Commun 14, 652 (2023). https://doi.org/10.1038/s41467-023-36161-4
Received:
Accepted:
Published:
DOI: https://doi.org/10.1038/s41467-023-36161-4
Comments
By submitting a comment you agree to abide by our Terms and Community Guidelines. If you find something abusive or that does not comply with our terms or guidelines please flag it as inappropriate.