Abstract
Malaria transmission-blocking vaccines (TBVs) target the development of Plasmodium parasites within the mosquito, with the aim of preventing malaria transmission from one infected individual to another. Different vaccine platforms, mainly protein-in-adjuvant formulations delivering the leading candidate antigens, have been developed independently and have reported varied transmission-blocking activities (TBA). Here, recombinant chimpanzee adenovirus 63, ChAd63 and modified vaccinia virus Ankara, MVA, expressing AgAPN1, Pfs230-C, Pfs25 and Pfs48/45 were generated. Antibody responses primed individually against all antigens by ChAd63 immunization in BALB/c mice were boosted by the administration of MVA expressing the same antigen. These antibodies exhibited a hierarchy of inhibitory activity against the NF54 laboratory strain of P. falciparum in Anopheles stephensi mosquitoes using the standard membrane feeding assay (SMFA), with anti-Pfs230-C and anti-Pfs25 antibodies giving complete blockade. The observed rank order of inhibition was replicated against P. falciparum African field isolates in A. gambiae in direct membrane feeding assays (DMFA). TBA achieved was IgG concentration dependent. This study provides the first head-to-head comparative analysis of leading antigens using two different parasite sources in two different vector species and can be used to guide selection of TBVs for future clinical development using the viral-vectored delivery platform.
Similar content being viewed by others
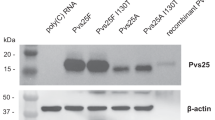
Introduction
Malaria is still one of the world’s major infectious diseases and exerts a devastating burden on global public health. The development of an effective vaccine against Plasmodium falciparum remains an important but elusive goal; to date, only low-level efficacies have been achieved by a handful of approaches in clinical studies1. The most advanced malaria vaccine candidate, currently in Phase III clinical trials, is based on the circumsporozoite protein (CSP) and aims to prevent infection of the vaccinated host2. Transmission-blocking vaccines (TBVs) target antigens expressed by the transmissible sexual-stages of the parasite, as well as those expressed by the mosquito and aim to reduce and/or block transmission to the vertebrate host3,4. Pre-clinical studies investigating TBVs have led to the development of a number of P. falciparum antigens as vaccine candidates of which Pfs230, Pfs25 and Pfs48/45 are well characterized. Different vaccine platforms utilizing these antigens have been used to raise antibodies with demonstrable transmission-blocking activity (TBA)5. However, to date only Pfs25 and its ortholog in P. vivax, Pvs25, have been tested in Phase Ia clinical trials6,7.
Pfs25 is expressed during macrogametogenesis within the mosquito midgut and persists throughout zygote, ookinete and early oocyst development. Pfs25 antigen-specific IgG demonstrate TBA and antibody titer correlates with ability to block parasite infectivity8,9,10. On the other hand, Pfs230 and Pfs48/45 are expressed on the surface of gametocytes within the human host and have a role in gamete-gamete recognition within the vector blood meal11,12. Individuals living in malaria endemic areas can naturally develop antibodies against these two antigens13,14,15,16.
Recently it has been shown that mosquito midgut ligands that mediate parasite invasion can also be targeted as TBV candidate antigens. Expressed midgut proteins of Anopheles stephensi and A. gambiae such as carboxypeptidase B4,17,18 and a 135 amino acid fragment of the A. gambiae midgut glycoprotein, alanyl aminopeptidase N1 (AgAPN1) have been developed as potential TBV candidates. Antibodies raised against this fragment of AgAPN1 have demonstrated TBA in studies using both P. falciparum and P. berghei parasites in two different anopheline species, A. gambiae and A. stephensi19.
Despite the lack of any reported comparison of TBA, Pfs230, Pfs25 and Pfs48/45 are currently considered the leading TBV candidate antigens. Their ability to induce a transmission-blocking response has been examined in different laboratories and the antigens have been expressed mainly as recombinant proteins produced in a variety of heterologous expression systems as well as delivered using vectored vaccine technologies20. The development of some TBVs, especially members of the six-cysteine family of proteins Pfs230 and Pfs48/45, has been significantly hampered by the difficulties associated with the expression of full-length, correctly folded native protein. The induction of transmission-blocking antibodies targeting these antigens is critically dependent on conformationally correct immunogens21,22. In the case of Pfs230, the region spanning amino acids 443-1132, referred to as region C (Pfs230-C), has been shown to induce the most potent TBA23. For Pfs48/45, codon harmonization of the native sequence has led to the expression of full-length protein, that induces antibodies giving greater than 95% inhibition of oocyst formation in both rodents and primates when formulated in the Montanide ISA-51 adjuvant24. Pfs25 protein has been used alone or conjugated to highly immunogenic carriers such as outer-membrane protein complex (OMPC) of Neisseria meningitidis serogroup B25. Pfs25 has also been delivered in a heterologous prime-boost regime using human adenovirus serotype 5 (HuAd5) and modified vaccinia virus Ankara (MVA) viral-vectors eliciting antibodies that exhibit TBA26.
Traditionally, viral vectors have been used in prime-boost regimes primarily for the induction of antigen-specific T cells, but HuAd5 and ChAd63, in a prime-boost regime with MVA, have also been used to induce functional antibodies against blood-stage malaria antigens in both pre-clinical and Phase I/IIa clinical studies27,28,29,30. Although potent as an antigen delivery vector, HuAd5 has not been favored for translation into the clinic due to the presence of neutralizing antibodies from pre-existing infections in individuals living in malaria endemic areas31. To circumvent this, chimpanzee adenoviruses (which are reported to have lower pre-existing neutralizing antibodies) have been used31. This human-compatible delivery system provides a robust and versatile platform to enable the testing and screening of TBV candidate antigens expressed in situ, while avoiding potential differences in potency caused by variable purification of expressed proteins.
In this study we have used the ChAd63-MVA heterologous prime-boost regime to compare four TBV candidate antigens head-to-head to identify antigen(s) for future clinical development in this viral vectored vaccination platform. We investigated AgAPN1, Pfs25, Pfs48/45 and region C of Pfs230 (Pfs230-C) and we assessed the ability of vaccine-induced IgG against these antigens to inhibit the development of P. falciparum oocysts within the mosquito, in order to guide prioritization of these candidates for clinical development in this delivery platform. We report that vaccine-induced IgG against Pfs230-C and Pfs25 completely blocked transmission of P. falciparum both the laboratory clone NF54 and field isolates from Burkina Faso.
Results:
Generation and expression of TBV candidate antigens in ChAd63 and MVA viral vectors
Recombinant ChAd63 and MVA vectors expressing Pfs25 were generated previously26. We designed and generated recombinant ChAd63 and MVA vaccines expressing three additional TBV candidate antigens: AgAPN1 based on the genome sequence of A. gambiae PEST strain; Pfs230-C and two versions of Pfs48/45 (Pfs48/45−NGln and Pfs48/45+NGln) based on the genomic sequence of the P. falciparum 3D7 clone (Table 1). For AgAPN1 and Pfs48/45 the signal peptide and glycosylphosphatidylinositol (GPI) anchor were not included in the construct. The inserts were used to generate recombinant ChAd63 and MVA expressing the individual antigens (Supplementary Information).
To test the expression of the antigens, pENTR4-LPTOS shuttle plasmid DNA expressing each individual antigen (under the control of the human cytomegalovirus (CMV) promoter32) was used to transfect HEK293 cells. Immunoblots of supernatants and cell lysates showed expression of the antigens at the expected size (AgAPN1 at 112 kDa, Pfs230-C at 83.5 kDa, Pfs25 at 18.8 kDa and Pfs48/45−NGln at 46 kDa)26 (Fig. 1). For Pfs48/45+NGln the molecular weight of the expressed protein was higher than expected (~60 kDa) possibly due to glycosylation. In Supplementary Fig. 1D when the supernatant was treated with Peptide-N-Glycosidase (optimized for the efficient release of N-linked oligosaccharides) the protein was expressed at the predicted size (46 kDa). Recombinant proteins were detected in supernatant for AgAPN1, Pfs230-C, Pfs25 and Pfs48/45+NGln suggesting that the incorporation of the tPA signal peptide leads to secretion of the antigen which is thought to be optimal for antibody induction33. Higher molecular weight bands were observed for Pfs230-C and Pfs48/45+NGln which could be dimers or a result of protein aggregation.
Expression of TBV candidate antigens in adherent HEK293 cells.
pENTR4-LPTOS shuttle plasmid DNA expressing (A) AgAPN1, (B) Pfs230-C, (C) Pfs25, (D) Pfs48/45−NGln and (E) Pfs48/45+NGln was used to transfect HEK293 cells and the supernatant and cell lysate were harvested. 5 μg protein was loaded per lane and after staining the western blots were developed for 5 min. The figure shows western blots using day70 anti-serum (pooled from 5 mice) collected after ChAd63-MVA vaccination against the respective antigens. Untransfected supernatant and cell lysate were used as negative controls. Lanes: 1- antigen-specific supernatant in reducing buffer; 2- antigen-specific supernatant in non-reducing buffer; 3- antigen-specific lysate in reducing buffer; 4- antigen-specific lysate in non-reducing buffer; 5- untransfected supernatant in non-reducing buffer; 6- untransfected supernatant in reducing buffer; 7- untransfected lysate in non-reducing buffer; 8- untransfected lysate in reducing buffer. A protein ladder is included in the figure for reference.
Heterologous prime-boost immunization induces IgG responses against TBV candidate antigens
We have previously shown that anti-Pfs25 antibodies, induced after vaccination with the HuAd5-MVA heterologous prime-boost vaccine regime, are functional and block the transmission of P. falciparum in a standard membrane feeding assay (SMFA)26 A pilot study was performed to test whether the ChAd63-MVA heterologous prime-boost regime was also able to induce antibodies to AgAPN1, Pfs230-C, Pfs48/45−NGln and Pfs48/45+NGln. Antigen-specific IgG was induced after the priming immunization with ChAd63 expressing the individual antigens, which was boosted significantly following the administration of the corresponding recombinant MVA (data not shown). Immune responses observed in the pilot study justified further testing of the longevity of vaccine-induced antibody responses. It has been previously shown that responses to Pfs25 eight weeks post-prime are similar whether primed with HuAd5 or ChAd6326.
IgG responses in this study were assessed at days 14 and 55 post-prime and day 14 post-boost (Fig. 2). Using Wilcoxon matched-pairs signed rank test the corresponding recombinant MVA significantly boosted the response to AgAPN1 (p = 0.008), Pfs230-C (p = 0.03) and Pfs48/45+NGln (p = 0.03). The increase in Pfs25 antibody response after the MVA boost was not statistically significant (p = 0.06). We have previously reported that MVA significantly boosts the antibody response to Pfs25 primed by HuAd526 and this is reproducible in other experiments when primed with ChAd63. In this particular experiment there was variability in the antibody response in the group of animals vaccinated against Pfs25 but we have repeated this experiment and seen less variability. The Pfs48/45−NGln (p = 0.09) antibody response was not significantly boosted by the MVA. When tested in the ELISA against recombinant protein produced using the wheat germ cell-free protein expression system, there was a consistent trend that the vaccine-induced IgG response against Pfs48/45+NGln compared to Pfs48/45−NGln (day 70) was higher. In order to assess the longevity of the antibody responses, the animals were monitored for ten and a half months post-boost. The responses were well maintained (no significant difference between day 70 and day 350 by Friedman test with Dunn’s multiple comparison test), for all the antigens except Pfs25.
Antigen-specific IgG responses in mice after immunization with ChAd63-MVA expressing TBV antigens.
BALB/c mice (n = 5/group) were primed with 1 × 108 infectious units (IFU) of ChAd63 (day 0) and boosted with 1 × 107 plaque forming units (PFU) of MVA (day 56) expressing the individual antigens AgAPN1, Pfs230-C, Pfs25, Pfs48/45−NGln and Pfs48/45+NGln. The figure shows the total IgG response measured by standardized ELISA on days 14, 55, 70 and thereafter every 28 days up to day 350. IgG responses were log-transformed and expressed as antibody units (AU) represented as mean ± SEM. The AU are not directly comparable between antigens, except for Pfs48/45 where the same coating antigen and ELISA method was used. Wilcoxon matched-pairs signed rank test was used to assess differences between day 55 and day 70 responses, whilst Friedman test with Dunn’s multiple comparison test was used to determine significance between day 70 and day 350. p ≤ 0.05 was considered significant (see Results text).
Vaccine-induced antibody responses recognize native parasite and mosquito proteins
To determine whether antibodies induced against Pfs230-C, Pfs25, Pfs48/45−NGln and Pfs48/45+NGln recognize the respective native parasite proteins, indirect immunofluorescence assays (IFA) were performed on parasite preparations (mature P. falciparum gametocytes or Pfs25DR3 P. berghei ookinetes) (Fig. 3). Surface staining of macrogametes with vaccine induced anti-Pfs230-C antibodies and ookinetes with anti-Pfs25 antibodies confirmed the ability of the antibodies to recognize native parasite protein. The anti-Pfs48/45+NGln antibodies stained the surface of exflagellating male gametes but no staining was observed with Pfs48/45−NGln antiserum (data not shown). Naïve and viral vector control serum (mice immunized with ChAd63-MVA expressing GFP) were used as negative controls and showed undetectable levels of staining (data not shown).
Reactivity of vaccine-induced antibodies against Pfs230-C, Pfs25 and Pfs48/45+NGln to native parasite antigen.
In vitro cultured Pfs25DR3 transgenic P. berghei ookinetes or P. falciparum NF54 microgametes and macrogametes were stained with purified total IgG from pooled serum (n = 5) against Pfs25 (top panel) or antisera against Pfs230-C (middle panel) and Pfs48/45+NGln (bottom panel). Antibody binding was detected by Alexa Fluor® 488-conjugated goat anti-mouse IgG (green) and the DNA was stained with DAPI (blue). Scale bars, 2 μm.
The anti-AgAPN1 serum recognized native antigen in an ELISA against midgut lysate from two strains of A. gambiae, Yaoundé and G3 (Fig. 4). Anti-AgAPN1 serum also recognized native AgAPN1 by western blot using midgut lysate from both A. gambiae Yaoundé (reducing and non-reducing conditions) and A. stephensi SDA500 (reducing condition) with similar banding patterns previously observed and described19 (Supplementary Fig. 1A). We also tested the anti-Pfs48/45−NGln and anti-Pfs48/45+NGln serum in a western blot using P. falciparum gametocyte lysate and they recognized a protein of 46 kDa (Supplementary Fig. 1B and C respectively).
Reactivity of vaccine-induced antibodies to A. gambiae midgut lysate.
BALB/c mice (n = 5) were vaccinated with ChAd63 expressing AgAPN1 (day 0) and boosted with the respective MVA (day 56). Antibody responses were measured on days 14, 55 and 70 by endpoint ELISA using midguts lysates obtained from A. gambiae Yaoundé and G3 strains. The data were log-transformed and are represented as mean ± SEM for the different time-points.
Inhibition of P. falciparum NF54 development in A. stephensi by vaccine-induced IgG
Vaccine-induced antibodies against Pfs230-C, Pfs25 and Pfs48/45 have shown varying TBA against P. falciparum depending on the antigen delivery system used24,34. Antibodies against AgAPN1 have been shown to inhibit the infectivity of both P. berghei and P. falciparum19,35. Here we performed a head-to-head comparison of the ability of antigen-specific IgG (induced against each antigen with a common vaccine platform) to reduce the intensity and prevalence of P. falciparum NF54 parasite infection in a laboratory-reared vector (A. stephensi) using SFMA. TBA of antibodies against Pfs230-C has been shown previously to be complement-dependent36,37 and thus all assays were performed in the presence of complement. To be able to distinguish antibody-specific inhibition from non-specific effects of mouse serum, total purified IgG was used.
IgG against Pfs230-C and Pfs25 showed complete inhibition of oocyst intensity and prevalence (p < 0.00001 for both) whereas anti-Pfs48/45+NGln IgG showed 99% inhibition of oocyst intensity and 85% inhibition of oocyst prevalence (p < 0.00001 for both) (Fig. 5). The IgG were tested in another independent SMFA and anti-Pfs230-C, Pfs25 and Pfs48/45+NGln all gave >89% of oocyst intensity (Supplementary Fig. 2). There was no statistically significant inhibition seen with anti-AgAPN1 (tested in two independent feeds) and anti-Pfs48/45−NGln IgG at the same IgG concentration.
Effect of mouse IgG induced by ChAd63-MVA immunization on P. falciparum NF54 parasite infectivity in A. stephensi mosquitoes.
Pooled day 70 serum (n = 5) was used to purify total IgG against each of the antigens. 655 μg/mL of each of the total IgG was mixed with P. falciparum NF54 cultured gametocytes and fed to A. stephensi mosquitoes (n = 20) in SMFA. Midguts were dissected 7 days post-feeding. Data points represent the number of oocysts in individual mosquitoes and the lines show the arithmetic mean (from one experiment). The table shows the number of mosquitoes dissected (N) per antigen, mean number of oocysts, percent inhibition of infection intensity and prevalence calculated relative to the IgG from mice immunized with vectors expressing GFP. GLMM with a zero-inflated negative binomial error structure was used to describe oocyst intensity whilst a binomial error structure was used to determine oocyst presence or absence. Uncertainty was generated using bootstrapping methodology. p ≤ 0.05 was considered significant.
Inhibition of African field isolates of P. falciparum in A. gambiae by vaccine-induced IgG
We also tested the IgG in a direct membrane feeding assay (DMFA) using P. falciparum parasites collected from gametocyte donors (children aged between 5 and 11 years) in Bobo-Dioulasso, Burkina Faso. This was tested using laboratory-adapted A. gambiae s.s, M molecular form, one of the predominant vectors for malaria transmission in this region. Although most TBV antigens have limited polymorphisms38, we wanted to assess whether the efficacy and the ranking of the antigens against P. falciparum NF54 would be replicated against field isolates in a second vector species.
Significant inhibition of oocyst intensity and prevalence consistent with the previous assay (Fig. 5) was seen with anti-Pfs230-C (complete inhibition, p < 0.00001 for both) and anti-Pfs25 (99% and 81.8% respectively, p < 0.00001 for both) IgG (Fig. 6). Anti-Pfs48/45+NGln IgG only significantly inhibited the oocyst intensity (45.5%, p = 0.007) and not prevalence in this experiment (Fig. 6). There was no significant inhibition seen with anti-AgAPN1 and anti-Pfs48/45−NGln IgG. The percent inhibition seen with anti-Pfs48/45+NGln IgG was lower than anti-Pfs230-C and anti-Pfs25 IgG.
Effect of mouse IgG induced by ChAd63-MVA immunization on infectivity of P. falciparum field isolate to A. gambiae mosquitoes.
The purified IgG (from pooled serum (n = 5)) for each of the antigens and gametocyte positive donor (BF001) RBCs were used in a DMFA. The gametocytaemia of the donor was 30 gametocytes per 1000 leukocytes and the ratio of male:female gametocytes was 1.44. The plasma from the gametocyte positive donor blood was replaced with 500 μg/mL IgG and immediately fed to A. gambiae mosquitoes. Midguts were dissected 7 days post-feeding. Data points represent the number of oocysts in individual mosquitoes and the lines show the arithmetic mean. The table shows the number of mosquitoes dissected (N) per antigen, mean number of oocysts, percent inhibition of infection intensity and prevalence calculated relative to the IgG from mice immunized with vectors expressing GFP. GLMM with a zero-inflated negative binomial error structure was used to describe oocyst intensity whilst a binomial error structure was used to determine oocyst presence or absence. Uncertainty was generated using bootstrapping methodology. p ≤ 0.05 was considered significant.
Anti-Pfs230-C and anti-Pfs25 IgG showed superior TBA (>99% inhibition of oocyst intensity) than the other antigens (Fig. 6). We thus investigated the effect of reducing the concentration of anti-Pfs230-C and anti-Pfs25 IgG in the membrane feeding assay (Figs 7 and 8). When tested at 250 μg/mL against a different gametocyte donor (BF002), both anti-Pfs230-C and anti-Pfs25 IgG still gave complete blockade. Significant inhibition of oocyst intensity and prevalence was also seen in this experiment with anti-Pfs230-C (p = 0.007 and p < 0.00001 respectively) and anti-Pfs25 (p < 0.00001 for both) IgG, with the same results observed at both 62.5 μg/mL and 125 μg/mL concentrations.
TBA of anti-Pfs230-C at different concentrations in DMFA. Membrane feeds were performed as described in Fig. 6 using a range of anti-Pfs230-C (7) and anti-Pfs25 (8) IgG concentrations (shown in brackets on the x-axis).
The gametocytaemia of the donor (BF002) was 10 gametocytes per 1000 leukocytes and the ratio of male:female gametocytes was 0.59. The IgG was tested with non-heat-inactivated AB+ serum as a source of complement. Data points represent the number of oocysts in individual mosquitoes and the lines show the arithmetic mean. The table shows the number of mosquitoes dissected (N) per antigen, mean number of oocysts, percent inhibition of infection intensity and prevalence calculated relative to the IgG from mice immunized with vectors expressing GFP. GLMM with a zero-inflated negative binomial error structure was used to describe oocyst intensity whilst a binomial error structure was used to determine oocyst presence or absence. Uncertainty was generated using bootstrapping methodology. p ≤ 0.05 was considered significant.
TBA of anti-Pfs25 IgG at different concentrations in DMFA. Membrane feeds were performed as described in Fig. 6 using a range of anti-Pfs230-C (7) and anti-Pfs25 (8) IgG concentrations (shown in brackets on the x-axis).
The gametocytaemia of the donor (BF002) was 10 gametocytes per 1000 leukocytes and the ratio of male:female gametocytes was 0.59. The IgG was tested with non-heat-inactivated AB+ serum as a source of complement. Data points represent the number of oocysts in individual mosquitoes and the lines show the arithmetic mean. The table shows the number of mosquitoes dissected (N) per antigen, mean number of oocysts, percent inhibition of infection intensity and prevalence calculated relative to the IgG from mice immunized with vectors expressing GFP. GLMM with a zero-inflated negative binomial error structure was used to describe oocyst intensity whilst a binomial error structure was used to determine oocyst presence or absence. Uncertainty was generated using bootstrapping methodology. p ≤ 0.05 was considered significant.
The anti-AgAPN1, anti-Pfs48/45−NGln and anti-Pfs48/45+NGln IgG were also tested in two further experiments using gametocytes from two different donors (BF003 and BF004). In this study anti-AgAPN1 IgG still showed no significant effect on oocyst prevalence in any of the experiments. However, anti-AgAPN1 IgG gave significant inhibition of oocyst intensity in one experiment (donor BF004, Control 2 in Supplementary Fig. 3) but this result was not replicated in any other feeds performed. The anti-Pfs48/45−NGln (82% and 78%, p = 0.01 and p < 0.0001 respectively) and anti-Pfs48/45+NGln (100%, p < 0.0001 for both) IgG showed significant inhibition in one assay (Control 2, Supplementary Fig. 3), whilst only anti-Pfs48/45−NGln IgG showed significant inhibition of oocyst intensity (55%, p = 0.02) in the other (Control 1, Supplementary Fig. 3). Control 2 had a lower oocyst number (8.5 oocysts) which could be due to the difference in the gametocytes, either amount ingested or sex ratio. The difference in inhibition could also be due to polymorphism in Pfs48/45, which has been reported previously38,39 or the number of parasite clones (multiplicity of infection), which might be an additional source of Pfs48/45 polymorphism in a single feed.
In light of the above results, we also expressed two smaller fragments of AgAPN1 using recombinant viral vectors; one corresponding to the N terminal fragment (amino acids 61-195) reported by Dinglasan et al.19 (AgAPN1 Nterm) and the other consisting of amino acids 20-303 based on the domain structure of AgAPN1 (AgAPN1 DomI). Total IgG was purified from sera (d70) from mice vaccinated with ChAd63 and MVA expressing AgAPN1, AgAPN1 DomI and AgAPN1 Nterm and tested in a SMFA at 750 μg/mL and 375 μg/mL (Supplementary Fig. 4). Anti-AgAPN1 and anti-AgAPN1 DomI IgG showed no significant effect on oocyst intensity and oocyst prevalence. Anti-AgAPN1 Nterm IgG gave significant inhibition of oocyst intensity (48%, p = 0.02) at 375 μg/mL but not 750 μg/mL, suggesting marginal TBA.
Discussion:
Here we describe a head-to-head comparison of the leading transmission-blocking vaccine candidate antigens using a human-compatible vaccine platform (heterologous prime-boost viral vectored delivery). TBV candidate antigens have been previously assessed individually for their ability to block parasite infectivity to mosquitoes using a wealth of different delivery platforms. In this comparative study, anti-Pfs230-C and anti-Pfs25 antibodies induced after vaccination with this viral vectored regime showed superior transmission-blocking activity compared to Pfs48/45 and AgAPN1 (each antigen construct (with the exception of Pfs48/45−Ngln) was tested a total of 2–4 times by SMFA and 3 times by DMFA with very similar results). IgG raised against Pfs230-C and Pfs25 have consistently shown better (>99%) TBA on both parasite infectivity and prevalence using both laboratory and field isolates of P. falciparum in A. stephensi and A. gambiae respectively. We have previously reported the use of this delivery system to induce functional antibodies to Pfs25 when expressed from a human adenovirus (HuAd5) and MVA26. This work provides additional evidence that the viral vectored delivery platform can induce functional antibodies against a range of candidate TBV antigens. This platform has been used successfully to induce functional antibodies against leading blood-stage malaria antigens in humans29,30,40 and used successfully to identify novel target antigens for further development41.
The development of certain TBV candidate antigens has been hampered by the inability to express full-length correctly folded proteins, like the sexual-stage cysteine-rich protein Pfs48/45 which has conformation-dependent target epitopes42,43. The viral vector delivery platform enabled us to express these antigens and induce antigen-specific antibodies. The ChAd63 vaccine primed a detectable antibody response that increased over time and was boosted after the administration of MVA expressing the respective antigen. These data are in accordance with previous findings where an 8 week interval between prime and boost was shown to induce high-titer antibodies against blood-stage malaria antigens and Pfs2526,28.
The antibody response is sustained for all the antigens (except Pfs25) using this delivery system, without the need for additional booster vaccinations after the initial prime-boost regime in mice. A sustained antibody response for at least one transmission season is likely to be essential to enable effective blockade in endemic areas. The decline in the antibody response to Pfs25 compared to the other antigens could be in part due to the inherent properties of the antigen. It has been reported previously that antibodies against Pfs25 decline to 50–70% of the peak response in mice after six months of follow up25. To address this, Pfs25 protein has been conjugated to several carrier proteins in an attempt to make it more immunogenic. A sustained antibody response was achieved in rhesus monkeys only after chemically conjugating Pfs25 to the OMPC of N. meningitidis serogroup B and adsorption to aluminum hydroxyphosphate25. A recent study has demonstrated that the induction of CD4+ T cells following an adjuvanted influenza vaccine predicted the persistence of the antibody response44 and several pre-clinical and clinical studies have shown that viral vectors induce strong T cell responses29,45. We did not assess the IgG subclass responses in this study but we have published previously that this viral vectored regime induces a mixed Th1/Th2 response26,45. The role of memory B cells which are important for a rapid recall response was also not assessed in this study, but viral vectored vaccination regimes have been shown to induce memory B cells in rhesus macaques27 and humans46. It will be important to further assess the role of CD4+ T cell help and memory B cells in the context of antibody responses to TBV candidate antigens, especially Pfs25 where antibody maintenance may be sub-optimal. In contrast to Pfs25, antibody responses to antigens such as Pfs230 and Pfs48/45 could potentially be boosted by natural infection and gametocyte exposure, but this requires experimental assessment. It has been shown in the P. berghei model that antibody responses induced by immunization with DNA encoding Pbs48/45 were boosted after a blood-stage challenge in mice47.
Our results suggest that there is a possible effect of N-glycosylation site substitution on conformation, immunogenicity and transmission-blocking activity of Pfs48/45. In previously published studies, expression of Pfs48/45 in vaccinia virus resulted in a glycosylated product that was considerably less immunogenic in mice resulting in a lowered ability to inhibit parasite infectivity to A. stephensi48,49. In contrast to the published data, the IgG against Pfs48/45−NGln (with N-glycosylation site substitutions) did not show any transmission blocking effect in the SMFA against P. falciparum NF54, but did recognize antigen in a Western blot. This could be due to the lower antibody response seen with this construct, or due to unintended alterations in functionally important, conformational B cell epitopes caused by amino acid substitutions. In only one of the DMFA did IgG against Pfs48/45−NGln show measurable TBA, but this was marginal and needs further investigation. Polymorphisms in Pfs48/45 have been reported in field isolates and this could explain the variability in TBA seen in the Burkina Faso studies38. The variability in TBA of antibodies against Pfs48/45 could also be dependent on the amount and sex ratio of gametocytes ingested. We conclude that further studies need to be done to understand the variability of TBA seen with IgG against Pfs48/45 and could include comparative assessment of the 10C region of Pfs48/4550. We are currently investigating further the impact of oocyst numbers in the control mosquitoes and antigen polymorphism on TBA in Burkina Faso. It is critical that such an approach be adopted for the screening of potential TBV candidate antigens as we have recently shown that vaccine efficacy needs to be tested against a range of parasite exposures to interpret laboratory results accurately51.
It was surprising that anti-AgAPN1 IgG induced by viral vectored prime-boost vaccination exhibited variable TBA. It has been shown previously that antibodies to an N-terminal fragment of AgAPN1 can block the development of both P. berghei and P. falciparum19,35. This difference in TBA could be due to the difference in construct design, although we have shown that the antibodies induced here recognize native antigen in both immunoblots and ELISA which provides evidence for recognition of the target antigen. It could also be due to presence or absence of O-linked glycosylation or due to the masking of transmission-blocking epitopes by other immunodominant epitopes52. This antigen remains an attractive candidate due to its effects on diverse Plasmodium species in different anopheline species but requires further investigation in this system.
It has been reported that antibodies against Pfs25 and Pfs28 have synergistic TBA when IgG against the two antigens are combined in an SMFA53 or when expressed as fusion protein in Saccharomyces cerevisiae54. Investigation of whether the same synergy could be achieved with anti-Pfs25 and Pfs230-C antibodies will be important and also ways to combine the two antigens in a single delivery platform. It will also be important in the future to determine the IC50 (concentration of antigen-specific antibody required to inhibit 50 percent of oocyst development)55 of the antibodies against Pfs230-C and Pfs25 against field isolates, in order to determine the concentration of IgG required for effective blockade in different transmission settings. The viral vector platform used in this study has a proven safety record in clinical trials targeting a range of pathogens30,56,57. The ability to induce antibody responses with functional activity against TBV candidate antigens with inhibitory effects of up to 100% observed for two antigens is very encouraging for further development of these candidate vaccines for clinical trials.
Methods:
Design and generation of recombinant viral vectored vaccines
Antigen sequences for AgAPN1, (amino acids (aa) 20–998; AGAP004809, PEST strain), Pfs230 (PF3D7_0209000), Pfs48/45 (aa 28–427; PF3D7_1346700) and Pfs25 (aa 22–192; PF3D7_1031000) were obtained from NCBI protein database. For Pfs230, only region C (aa 443–1132, Pfs230-C) against which antibodies have been shown previously to inhibit parasite development was used58,59. All putative N-glycosylation sites were changed from Asn-Xaa-Ser/Thr to Gln-Xaa-Ser/Thr. For Pfs48/45 two different constructs were designed; one with all seven N-glycosylation sites43 substituted (Pfs48/45−NGln) and the other with the native amino acid sequence (Pfs48/45+NGln). In addition, two fragments of AgAPN1 were designed corresponding to amino acids 61–195 (AgAPN1 Nterm) and amino acids 20–303 (AgAPN1 DomI). The antigen sequences were codon optimized for expression in humans (GeneArt® Life Technologies, Germany) and the predicted native signal peptide for each antigen was replaced with the human tissue plasminogen activator (tPA) signal peptide28. The recombinant viral vaccines were generated as described in Supplementary Information.
Animal studies and vaccinations
Age-matched female BALB/c mice (Harlan, UK), housed in specific-pathogen free environments, were vaccinated via the intramuscular (i.m.) route using a heterologous prime-boost regime. To determine whether AgAPN1, Pfs230-C, Pfs48/45−NGln and Pfs48/45+NGln were immunogenic when administered in this delivery system, which has been previously shown to elicit anti-Pfs25 specific antibodies26, a pilot study was undertaken. In all experiments, animals were vaccinated with a ChAd63 (day 0) priming dose of 1 × 108 IFU and boosted with MVA (day 56) at 1 × 107 PFU expressing the individual test antigens. Control vaccinations were performed with ChAd63 and MVA expressing green fluorescent protein (GFP). Vaccines were prepared in sterile endotoxin-free PBS (Invitrogen, UK). Vaccine-induced antibody responses were assessed on days 14, 55 and 70 and then every month for ten and a half months. All animal experiments and procedures were performed according to the UK Animals (Scientific Procedures) Act Project Licence (PPL 30/2414 and 30/2889) and approved by the Oxford University Local Ethical Review Committee.
Western Blot Analysis
To determine the expression of the recombinant antigens expressed by the constructs in mammalian cells, 1 × 107 cells/mL HEK293 cells were seeded onto 6 well plates and transfected with pENTR™ (Invitrogen, UK) entry plasmid (containing the tetracycline operator in proximity to the TATA box, pENTR™4LPTOS)41) using Lipofectamine™ 2000 (Invitrogen, UK). Cells were incubated overnight at 37 °C and 5% CO2. Supernatant and cell lysate were harvested in non-reducing and reducing, SDS-PAGE loading buffers and boiled at 95 °C for 5 min before running on 8 or 12% polyacrylamide gels (Thermo Scientific, UK) (5 μg/lane). Proteins were transferred onto 0.45 μm nitrocellulose membranes, which were then blocked in 3% BSA/PBS overnight, washed twice with PBS/0.05% Tween®20 (PBS/T) and incubated with sera (pooled d70 serum from all mice (n = 5) immunized with the respective antigens) diluted 1:100 in 3% BSA/PBS for 1 h. The blots were then washed and incubated for 1 h with donkey anti-mouse IgG AP-conjugated antibody (Jackson ImmunoResearch Laboratories, Inc.) diluted at 1:5000, in 3% BSA/PBS. After repeating the washing procedure, the protein bands were detected using BCIP/NBT alkaline phosphatase substrate (Sigma, UK) and after 5 min the images were scanned using Canon Canoscan LiDe200. Color plus Pre-stained Protein ladders (NEB, UK) were used to estimate the relative protein mobility.
ELISAs
To detect antigen-specific IgG antibodies induced by vaccination, ELISAs were performed as previously described10,60. The recombinant proteins for AgAPN1, Pfs230-C and Pfs48/45 were produced using the wheat-germ cell free system61 and the Pfs25 protein antigen was provided by Dr Yimin Wu (NIH, USA). A standard reference serum was created using pooled day 70 vaccinated mouse serum with high titers to the respective antigens. The standard reference was used on a standardized ELISA to determine the ELISA units, expressed as antibody units (AU), for each individual antigen. For Pfs25 ELISAs, a previously reported standard serum was used10,25. A negative control (pooled serum from mice immunized with ChAd63 and MVA expressing GFP) was included and the OD values for the negative control were less than 0.15 for all the plates tested.
Indirect Immunofluorescence Assay
P. falciparum gametocytes were cultured as described previously10 and pelleted. For gametocyte preparations, cultures were split and half were activated in ookinete media62, following which samples were fixed for 10 min in 4% PFA in PBS. The non-activated and activated preparations were then pipetted onto poly-Lysine coated multiwell slides, air dried and allowed to settle for 12 h, to allow the adherence of parasites and red blood cells (RBCs) to slides. Slides were then washed three times in Tris-buffered saline (TBS) and blocked using 10% goat serum (Sigma, UK) in 1% BSA/PBS for 1 h. The slides were incubated for 1 h with day 70 mouse antisera (Pfs230-C, Pfs48/45−NGln and Pfs48/45+NGln) or purified total IgG (2.5 mg/ml for Pfs25) diluted 1:100 in 1%BSA/PBS. After washing three times in TBS, goat anti-mouse IgG conjugated to Alexa Fluor 488 (Invitrogen, UK; 1:500 in 1% BSA/PBS) was added for 1 h, washed off in TBS and mounted with Vectashield/DAPI (Vector Labs, UK). The slides were visualized under x100 objective magnification using a fluorescence microscope (Leica DMI 3000 B, Leica Microsystems, UK). To assess antibody reactivity with native Pfs25 on ookinetes, Pfs25DR3 transgenic P. berghei parasites described previously26 expressing Pfs25 were used. Phenylhydrazine (6 μg/mL in PBS; Sigma, UK) treated female TO (Harlan, UK) mice were injected with 1 × 107 Pfs25DR3 parasitized RBCs (pRBCs). Parasitized blood was harvested from the infected mouse and added 1:25 to ookinete media and left overnight at 19 °C. After 24 h, smears were fixed in 4% PFA in PBS after air-drying. Thereafter the procedure was carried out as outlined above.
IgG Purification
Total IgG was purified from day 70 pooled mouse sera (equal volume of serum from all mice in a group was pooled irrespective of individual antibody titer) from test and control (vectors expressing GFP) groups as described previously55 using Protein G columns (Pierce, USA). Briefly, Protein G columns were equilibrated with binding buffer (Immunopure IgG, Pierce, USA) after which a 1:1 mixture of sera and binding buffer was allowed to flow through under gravity, washed and eluted with elution buffer (Immunopure IgG, Pierce, USA). The eluted fraction was collected in 1 M Tris-HCl (pH 9.0, Teknova, USA) and transferred for buffer exchange to Amicon centrifugal filters (Millipore, USA) using PBS. The eluent was concentrated in PBS (Invitrogen, UK) and filtered using a 0.22 μm Millipore Ultrafree sterile centrifugal unit. Total protein was quantified using a NanoDrop spectrophotometer at 280 nm and adjusted to either 2.5 mg/mL, 3.5 mg/mL or 4 mg/mL.
Transmission-Blocking Assays
The ability of vaccine-induced antibodies to block the development of P. falciparum strain NF54 was assayed using gametocyte cultures as previously described10. In the case of cultured NF54 parasites, the percentage of mature Stage V gametocytes was adjusted to 0.15% ± 0.05% and the male-female ratio is stable (almost always 1 male : 2–3 female). These were mixed with purified IgG (diluted at 1/5.34 from adjusted total concentration of either 2.5 mg/mL, 3.5 mg/mL or 4 mg/mL giving a testing dilution of either 0.500 mg/mL, 0.655 mg/mL or 0.75 mg/mL respectively) and then fed to 4–6 day old starved female A. stephensi (SDA 500) via a parafilm® membrane. The purified IgG was diluted in non-heat-inactivated human AB+ sera. Purified IgG from a group of mice vaccinated with viral vectors expressing GFP was used as negative control. Infected mosquitoes were then maintained at 26 °C and 80% relative humidity. After 7 days midguts from twenty mosquitoes were dissected, oocysts counted and the number of infected mosquitoes recorded. Percent reduction in infection intensity and prevalence were calculated relative to the control.
To determine the TBA against field isolates of P. falciparum, children aged between 5–11 years in Bobo-Dioulasso, Burkina Faso, were screened for the presence of asexual- and sexual-stage P. falciparum parasites by thick blood smears. Informed consent from parents or guardians was obtained for children positive for gametocytes (Protocol 003-2009/CE-CM, Centre Muraz institutional ethical committee). 10 mL blood was drawn in heparinized tubes to obtain gametocytes. The plasma from the gametocyte positive donor blood was replaced by AB+ serum from a European donor, the purified IgG were added at 0.500 mg/mL in the final mixture and immediately fed to A. gambiae via a parafilm® membrane. Thereafter the procedure was carried out as outlined above. All SMFA and DMFA experiments were performed using non-heat-inactivated AB+ serum as a source of complement.
Statistical Analysis
For the ELISA data, Wilcoxon matched-pairs signed rank test was used to determine statistical significance between pre- and post-boost (day 55 and day 70) response and Mann-Whitney test between Pfs48/45−NGln and Pfs48/45+NGln (day 70 responses). To determine significance of the longevity of the vaccine-induced antibody response, a Friedman test with Dunn’s correction was used to assess differences between time-points for each individual test antigen. Ability of vaccine-induced antibodies to inhibit oocyst intensity and prevalence of P. falciparum in transmission-blocking assays was assessed using generalized linear mixed models (GLMM) according to previously published methods51. A zero-inflated negative binomial error structure was used to describe oocyst intensity whilst a binomial error structure was used to determine oocyst presence or absence. Models were compared using a likelihood ratio test and confidence intervals generated using bootstrapping methodology. Significance was taken as p < 0.05.
Additional Information
How to cite this article: Kapulu, M. C. et al. Comparative Assessment of Transmission-Blocking Vaccine Candidates against Plasmodium falciparum. Sci. Rep. 5, 11193; doi: 10.1038/srep11193 (2015).
References
Birkett, A. J., Moorthy, V. S., Loucq, C., Chitnis, C. E. & Kaslow, D. C. Malaria vaccine R&D in the Decade of Vaccines: breakthroughs, challenges and opportunities. Vaccine 31 Suppl 2, B233–243 (2013).
Agnandji, S. T. et al. A phase 3 trial of RTS,S/AS01 malaria vaccine in African infants. N Engl J Med 367, 2284–2295 (2012).
Carter, R. Transmission blocking malaria vaccines. Vaccine 19, 2309–2314 (2001).
Lavazec, C. & Bourgouin, C. Mosquito-based transmission blocking vaccines for interrupting Plasmodium development. Microbes and infection / Institut Pasteur 10, 845–849 (2008).
Carter, R., Mendis, K., Miller, L., Molineaux, L. & Saul, A. Malaria transmission-blocking vaccines--how can their development be supported? Nat Med 6, 241–244 (2000).
Malkin, E. M. et al. Phase 1 vaccine trial of Pvs25H: a transmission blocking vaccine for Plasmodium vivax malaria. Vaccine 23, 3131–3138 (2005).
Wu, Y. et al. Phase 1 trial of malaria transmission blocking vaccine candidates Pfs25 and Pvs25 formulated with montanide ISA 51. PLoS One 3, e2636 (2008).
Kaslow, D. et al. A vaccine candidate from the sexual stage of human malaria that contains EGF-like domains. Nature 333, 74–76 (1988).
Barr, P. et al. Recombinant Pfs25 protein of Plasmodium falciparum elicits malaria transmission-blocking immunity in experimental animals. J Exp Med 174, 1203–1208 (1991).
Miura, K. et al. Transmission-blocking activity induced by malaria vaccine candidates Pfs25/Pvs25 is a direct and predictable function of antibody titer. Malar J 6, 107 (2007).
Eksi, S. et al. Malaria transmission-blocking antigen, Pfs230, mediates human red blood cell binding to exflagellating male parasites and oocyst production. Mol Microbiol 61, 991–998 (2006).
van Dijk, M. R. et al. A central role for P48/45 in malaria parasite male gamete fertility. Cell 104, 153–164 (2001).
Bousema, T. et al. The dynamics of naturally acquired immune responses to Plasmodium falciparum sexual stage antigens Pfs230 & Pfs48/45 in a low endemic area in Tanzania. PLoS One 5, e14114 (2010).
Bousema, J. et al. A longitudinal study of immune responses to Plasmodium falciparum sexual stage antigens in Tanzanian adults. Parasite Immunol 29, 309–317 (2007).
Healer, J., McGuinness, D., Carter, R. & Riley, E. Transmission-blocking immunity to Plasmodium falciparum in malaria-immune individuals is associated with antibodies to the gamete surface protein Pfs230. Parasitology 119 (Pt 5), 425–433 (1999).
Roeffen, W. et al. Association between anti-Pfs48/45 reactivity and P. falciparum transmission-blocking activity in sera from Cameroon. Parasite Immunol 18, 103–109 (1996).
Lavazec, C. et al. Carboxypeptidases B of Anopheles gambiae as Targets for a Plasmodium falciparum Transmission-Blocking Vaccine. Infect. Immun. 75, 1635–1642 (2007).
Raz, A., Dinparast Djadid, N. & Zakeri, S. Molecular characterization of the carboxypeptidase B1 of Anopheles stephensi and its evaluation as a target for transmission-blocking vaccines. Infection and immunity 81, 2206–2216 (2013).
Dinglasan, R. R. et al. Disruption of Plasmodium falciparum development by antibodies against a conserved mosquito midgut antigen. Proc Natl Acad Sci U S A 104, 13461–13466 (2007).
Nikolaeva, D., Draper, S. J. & Biswas, S. Toward the development of effective transmission-blocking vaccines for malaria. Expert review of vaccines, 1–28, doi:10.1586/14760584.2015.993383 (2015).
van Dijk, M. R. et al. Three members of the 6-cys protein family of Plasmodium play a role in gamete fertility. PLoS Pathog 6, e1000853 (2010).
Williamson, K. C., Fujioka, H., Aikawa, M. & Kaslow, D. C. Stage-specific processing of Pfs230, a Plasmodium falciparum transmission-blocking vaccine candidate. Mol Biochem Parasitol 78, 161–169 (1996).
Williamson, K. C., Keister, D. B., Muratova, O. & Kaslow, D. C. Recombinant Pfs230, a Plasmodium falciparum gametocyte protein, induces antisera that reduce the infectivity of Plasmodium falciparum to mosquitoes. Mol Biochem Parasitol 75, 33–42 (1995).
Chowdhury, D. R., Angov, E., Kariuki, T. & Kumar, N. A potent malaria transmission blocking vaccine based on codon harmonized full length Pfs48/45 expressed in Escherichia coli. PLoS One 4, e6352 (2009).
Wu, Y. et al. Sustained high-titer antibody responses induced by conjugating a malarial vaccine candidate to outer-membrane protein complex. Proc Natl Acad Sci USA 103, 18243–18248 (2006).
Goodman, A. L. et al. A viral vectored prime-boost immunization regime targeting the malaria Pfs25 antigen induces transmission-blocking activity. PLoS One 6, e29428 (2011).
Draper, S. J. et al. Enhancing blood-stage malaria subunit vaccine immunogenicity in rhesus macaques by combining adenovirus, poxvirus and protein-in-adjuvant vaccines. J Immunol 185, 7583–7595 (2010).
Draper, S. J. et al. Effective induction of high-titer antibodies by viral vector vaccines. Nat Med 14, 819–821 (2008).
Sheehy, S. H. et al. Phase Ia clinical evaluation of the Plasmodium falciparum blood-stage antigen MSP1 in ChAd63 and MVA vaccine vectors. Mol Ther 19, 2269–2276 (2011).
Sheehy, S. H. et al. ChAd63-MVA-vectored blood-stage malaria vaccines targeting MSP1 and AMA1: assessment of efficacy against mosquito bite challenge in humans. Mol Ther 20, 2355–2368 (2012).
Dudareva, M. et al. Prevalence of serum neutralizing antibodies against chimpanzee adenovirus 63 and human adenovirus 5 in Kenyan children, in the context of vaccine vector efficacy. Vaccine 27, 3501–3504 (2009).
Sridhar, S. et al. Single-Dose Protection against Plasmodium berghei by a Simian Adenovirus Vector Using a Human Cytomegalovirus Promoter Containing Intron A. J. Virol. 82, 3822–3833 (2008).
Biswas, S. et al. Transgene Optimization, Immunogenicity and In Vitro Efficacy of Viral Vectored Vaccines Expressing Two Alleles of Plasmodium falciparum AMA1. PLoS One 6, e20977 (2011).
Milek, R. L., Stunnenberg, H. G. & Konings, R. N. Assembly and expression of a synthetic gene encoding the antigen Pfs48/45 of the human malaria parasite Plasmodium falciparum in yeast. Vaccine 18, 1402–1411 (2000).
Mathias, D. K. et al. Expression, immunogenicity, histopathology and potency of a mosquito-based malaria transmission-blocking recombinant vaccine. Infect. Immun. 80, 1606–1614 (2012).
Healer, J. et al. Complement-mediated lysis of Plasmodium falciparum gametes by malaria-immune human sera is associated with antibodies to the gamete surface antigen Pfs230. Infect. Immun. 65, 3017–3023 (1997).
Read, D. et al. Transmission-blocking antibodies against multiple, non-variant target epitopes of the Plasmodium falciparum gamete surface antigen Pfs230 are all complement-fixing. Parasite Immunol 16, 511–519 (1994).
Niederwieser, I., Felger, I. & Beck, H. P. Limited polymorphism in Plasmodium falciparum sexual-stage antigens. Am J Trop Med Hyg 64, 9–11 (2001).
Escalante, A. A., Lal, A. A. & Ayala, F. J. Genetic polymorphism and natural selection in the malaria parasite Plasmodium falciparum. Genetics 149, 189–202 (1998).
Sheehy, S. H. et al. Phase Ia clinical evaluation of the safety and immunogenicity of the Plasmodium falciparum blood-stage antigen AMA1 in ChAd63 and MVA vaccine vectors. PLoS One 7, e31208 (2012).
Douglas, A. D. et al. The blood-stage malaria antigen PfRH5 is susceptible to vaccine-inducible cross-strain neutralizing antibody. Nat Commun 2, 601 (2011).
Outchkourov, N. S. et al. Correctly folded Pfs48/45 protein of Plasmodium falciparum elicits malaria transmission-blocking immunity in mice. Proc Natl Acad Sci USA 105, 4301–4305 (2008).
Kocken, C. H. et al. Cloning and expression of the gene coding for the transmission blocking target antigen Pfs48/45 of Plasmodium falciparum. Mol Biochem Parasitol 61, 59–68 (1993).
Galli, G. et al. Adjuvanted H5N1 vaccine induces early CD4+ T cell response that predicts long-term persistence of protective antibody levels. Proc Natl Acad Sci USA 106, 3877–3882 (2009).
Douglas, A. D. et al. Tailoring subunit vaccine immunogenicity: maximizing antibody and T cell responses by using combinations of adenovirus, poxvirus and protein-adjuvant vaccines against Plasmodium falciparum MSP1. Vaccine 28, 7167–7178 (2010).
Elias, S. C. et al. Analysis of human B-cell responses following ChAd63-MVA MSP1 and AMA1 immunization and controlled malaria infection. Immunology 141, 628–644 (2014).
Haddad, D., Maciel, J. & Kumar, N. Infection with Plasmodium berghei boosts antibody responses primed by a DNA vaccine encoding gametocyte antigen Pbs48/45. Infect. Immun. 74, 2043–2051 (2006).
Milek, R. L. et al. Plasmodium falciparum: heterologous synthesis of the transmission-blocking vaccine candidate Pfs48/45 in recombinant vaccinia virus-infected cells. Exp Parasitol 90, 165–174 (1998).
Bushkin, G. G. et al. Suggestive evidence for Darwinian Selection against asparagine-linked glycans of Plasmodium falciparum and Toxoplasma gondii. Eukaryot Cell 9, 228–241 (2010).
Theisen, M. et al. A multi-stage malaria vaccine candidate targeting both transmission and asexual parasite life-cycle stages. Vaccine 32, 2623–2630 (2014).
Churcher, T. S. et al. Measuring the blockade of malaria transmission--an analysis of the Standard Membrane Feeding Assay. Int J Parasitol 42, 1037–1044 (2012).
Armistead, J. S. et al. Antibodies to a single, conserved epitope in Anopheles APN1 inhibit universal transmission of Plasmodium falciparum and Plasmodium vivax malaria. Infection and immunity 82, 818–829 (2014).
Duffy, P. E. & Kaslow, D. C. A novel malaria protein, Pfs28 and Pfs25 are genetically linked and synergistic as falciparum malaria transmission-blocking vaccines. Infect. Immun. 65, 1109–1113 (1997).
Gozar, M. M., Price, V. L. & Kaslow, D. C. Saccharomyces cerevisiae-secreted fusion proteins Pfs25 and Pfs28 elicit potent Plasmodium falciparum transmission-blocking antibodies in mice. Infect. Immun. 66, 59–64 (1998).
Cheru, L. et al. The IC(50) of anti-Pfs25 antibody in membrane-feeding assay varies among species. Vaccine 28, 4423–4429 (2010).
Berthoud, T. K. et al. Potent CD8+ T-cell immunogenicity in humans of a novel heterosubtypic influenza A vaccine, MVA-NP+M1. Clin Infect Dis 52, 1–7 (2011).
Tameris, M. D. et al. Safety and efficacy of MVA85A, a new tuberculosis vaccine, in infants previously vaccinated with BCG: a randomised, placebo-controlled phase 2b trial. Lancet 381, 1021–1028 (2013).
Bustamante, P. J. et al. Differential ability of specific regions of Plasmodium falciparum sexual-stage antigen, Pfs230, to induce malaria transmission-blocking immunity. Parasite Immunol 22, 373–380 (2000).
Tachibana, M. et al. N-terminal prodomain of Pfs230 synthesized using a cell-free system is sufficient to induce complement-dependent malaria transmission-blocking activity. Clinical and vaccine immunology : CVI 18, 1343–1350 (2011).
Miura, K. et al. Development and characterization of a standardized ELISA including a reference serum on each plate to detect antibodies induced by experimental malaria vaccines. Vaccine 26, 193–200 (2008).
Tsuboi, T. et al. Wheat germ cell-free system-based production of malaria proteins for discovery of novel vaccine candidates. Infection and immunity 76, 1702–1708 (2008).
Blagborough, A. M. & Sinden, R. E. Plasmodium berghei HAP2 induces strong malaria transmission-blocking immunity in vivo and in vitro. Vaccine 27, 5187–5194 (2009).
Acknowledgements
We thank J. Furze and the Jenner Institute Vector Core Facility for generating the recombinant viral vectors. We would also like to thank S. Moretz, A. Diouf, G. Tullo and B. Deng for performing the SMFA; Dr Y. Wu for providing the recombinant Pfs25 protein and reference serum used in ELISAs; and Dr G. Christophides for providing the mosquitoes for midgut ELISA. As. MCK was a recipient of a Commonwealth Scholarship. SB is a NDM Leadership Fellow and a Junior Research Fellow of St. Catherine’s College at the University of Oxford. This work was funded by a Wellcome Trust grant (WT100157MA) to AVSH. SJD holds a MRC Career Development Fellowship (grant number G1000527) and is a Lister Institute Research Prize Fellow. S.C.G., S.J.D. and A.V.S.H. are Jenner Investigators. AC received funding from the European Community’s Seventh Framework Program (FP7/2007–2013) under grant agreements numbers 242095 and 223736 and DFD received an IRD fellowship. AMB is funded by Fraunhofer Institute and Medicines for Malaria Venture. SMFA work at NIH MFA Evaluation Centre was supported in part by the intramural program of the NIAID, NIH and in part by the PATH Malaria Vaccine Initiative. TT was supported in part by MEXT/JSPS KAKENHI grant numbers 23117008 and 23406007. ALG was funded by a MRC clinical training fellowship grant number G0600424.
Author information
Authors and Affiliations
Contributions
M.C.K. performed the experiments, western blots and statistical analysis on the immunogenicity data. D.F.D., I.S. and A.C. performed the feeding assays in Burkina Faso. K.M. and C.A.L. performed the feeding assays against P. falciparum NF54 at the NIH MFA Reference Facility. T.S.C. performed statistical analysis on all the feeding assays. M.C.K., A.M.B. and U.S. cultured P. falciparum NF54 and performed the immunofluorescence assays. S.J.D. and A.L.G. designed the Pfs25 construct. Y.L. and D.N. helped with the western blots. A.N. provided the ChAd63 viral vector. T.T. produced the recombinant proteins used in ELISAs. A.R.W., A.V.T., M.G.C., S.J.D. and S.C.G. provided advice on study design. M.C.K., R.E.S., A.V.S.H. and S.B. designed the study. M.C.K. and S.B. wrote the paper. All authors reviewed the manuscript.
Ethics declarations
Competing interests
The authors declare no competing financial interests.
Electronic supplementary material
Rights and permissions
This work is licensed under a Creative Commons Attribution 4.0 International License. The images or other third party material in this article are included in the article’s Creative Commons license, unless indicated otherwise in the credit line; if the material is not included under the Creative Commons license, users will need to obtain permission from the license holder to reproduce the material. To view a copy of this license, visit http://creativecommons.org/licenses/by/4.0/
About this article
Cite this article
Kapulu, M., Da, D., Miura, K. et al. Comparative Assessment of Transmission-Blocking Vaccine Candidates against Plasmodium falciparum. Sci Rep 5, 11193 (2015). https://doi.org/10.1038/srep11193
Received:
Accepted:
Published:
DOI: https://doi.org/10.1038/srep11193
This article is cited by
-
Design of a stabilized non-glycosylated Pfs48/45 antigen enables a potent malaria transmission-blocking nanoparticle vaccine
npj Vaccines (2023)
-
Vector microbiota manipulation by host antibodies: the forgotten strategy to develop transmission-blocking vaccines
Parasites & Vectors (2022)
-
Elucidating functional epitopes within the N-terminal region of malaria transmission blocking vaccine antigen Pfs230
npj Vaccines (2022)
-
Monoclonal antibodies block transmission of genetically diverse Plasmodium falciparum strains to mosquitoes
npj Vaccines (2021)
-
Malaria transmission-blocking conjugate vaccine in ALFQ adjuvant induces durable functional immune responses in rhesus macaques
npj Vaccines (2021)
Comments
By submitting a comment you agree to abide by our Terms and Community Guidelines. If you find something abusive or that does not comply with our terms or guidelines please flag it as inappropriate.