Abstract
The mammalian circadian clock is built on a molecular feedback loop in which the Period (PER) proteins, acting in a large, poorly understood complex, repress Clock–Bmal1, the transcription factor driving their expression. We found that mouse PER complexes include the histone methyltransferase HP1γ–Suv39h. PER proteins recruited HP1γ–Suv39h to the Per1 and Per2 promoters, and HP1γ–Suv39h proved important for circadian di- and trimethylation of histone H3 Lys9 (H3K9) at the Per1 promoter, feedback repression and clock function. HP1γ–Suv39h was recruited to the Per1 and Per2 promoters ~4 h after recruitment of HDAC1, a PER-associated protein previously implicated in clock function and H3K9 deacetylation at the Per1 promoter. PER complexes containing HDAC1 or HP1γ–Suv39h appeared to be physically separable. Circadian clock negative feedback by the PER complex thus involves dynamic, ordered recruitment of repressive chromatin modifiers to DNA-bound Clock–Bmal1.
This is a preview of subscription content, access via your institution
Access options
Subscribe to this journal
Receive 12 print issues and online access
$189.00 per year
only $15.75 per issue
Buy this article
- Purchase on Springer Link
- Instant access to full article PDF
Prices may be subject to local taxes which are calculated during checkout






Similar content being viewed by others
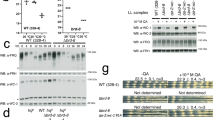
References
Mohawk, J.A., Green, C.B. & Takahashi, J.S. Central and peripheral circadian clocks in mammals. Annu. Rev. Neurosci. 35, 445–462 (2012).
Takahashi, J.S., Hong, H.K., Ko, C.H. & McDearmon, E.L. The genetics of mammalian circadian order and disorder: implications for physiology and disease. Nat. Rev. Genet. 9, 764–775 (2008).
Dibner, C., Schibler, U. & Albrecht, U. The mammalian circadian timing system: organization and coordination of central and peripheral clocks. Annu. Rev. Physiol. 72, 517–549 (2010).
Schibler, U., Ripperger, J. & Brown, S.A. Peripheral circadian oscillators in mammals: time and food. J. Biol. Rhythms 18, 250–260 (2003).
Yamazaki, S. et al. Resetting central and peripheral circadian oscillators in transgenic rats. Science 288, 682–685 (2000).
Balsalobre, A., Damiola, F. & Schibler, U. A serum shock induces circadian gene expression in mammalian tissue culture cells. Cell 93, 929–937 (1998).
Lamia, K.A., Storch, K.F. & Weitz, C.J. Physiological significance of a peripheral tissue circadian clock. Proc. Natl. Acad. Sci. USA 105, 15172–15177 (2008).
Durgan, D.J. et al. The circadian clock within the cardiomyocyte is essential for responsiveness of the heart to fatty acids. J. Biol. Chem. 281, 24254–24269 (2006).
Jeyaraj, D. et al. Circadian rhythms govern cardiac repolarization and arrhythmogenesis. Nature 483, 96–99 (2012).
Marcheva, B. et al. Disruption of the clock components CLOCK and BMAL1 leads to hypoinsulinaemia and diabetes. Nature 466, 627–631 (2010).
Sadacca, L.A., Lamia, K.A., deLemos, A.S., Blum, B. & Weitz, C.J. An intrinsic circadian clock of the pancreas is required for normal insulin release and glucose homeostasis in mice. Diabetologia 54, 120–124 (2011).
Ouyang, Y., Andersson, C.R., Kondo, T., Golden, S.S. & Johnson, C.H. Resonating circadian clocks enhance fitness in cyanobacteria. Proc. Natl. Acad. Sci. USA 95, 8660–8664 (1998).
Brown, S.A. et al. PERIOD1-associated proteins modulate the negative limb of the mammalian circadian oscillator. Science 308, 693–696 (2005).
Padmanabhan, K., Robles, M.S., Westerling, T. & Weitz, C.J. Feedback regulation of transcriptional termination by the mammalian circadian clock PERIOD complex. Science 337, 599–602 (2012).
Kume, K. et al. mCRY1 and mCRY2 are essential components of the negative limb of the circadian clock feedback loop. Cell 98, 193–205 (1999).
Sangoram, A.M. et al. Mammalian circadian autoregulatory loop: a timeless ortholog and mPer1 interact and negatively regulate CLOCK–BMAL1-induced transcription. Neuron 21, 1101–1113 (1998).
Griffin, E.A. Jr., Staknis, D. & Weitz, C.J. Light-independent role of CRY1 and CRY2 in the mammalian circadian clock. Science 286, 768–771 (1999).
Siepka, S.M. et al. Circadian mutant Overtime reveals F-box protein FBXL3 regulation of Cryptochrome and Period gene expression. Cell 129, 1011–1023 (2007).
Godinho, S.I. et al. The after-hours mutant reveals a role for Fbxl3 in determining mammalian circadian period. Science 316, 897–900 (2007).
Asher, G. et al. SIRT1 regulates circadian clock gene expression through PER2 deacetylation. Cell 134, 317–328 (2008).
Busino, L. et al. SCFFbxl3 controls the oscillation of the circadian clock by directing the degradation of cryptochrome proteins. Science 316, 900–904 (2007).
Solt, L.A. et al. Regulation of circadian behaviour and metabolism by synthetic REV-ERB agonists. Nature 485, 62–68 (2012).
Cho, H. et al. Regulation of circadian behaviour and metabolism by REV-ERB-α and REV-ERB-β. Nature 485, 123–127 (2012).
Rey, G. et al. Genome-wide and phase-specific DNA-binding rhythms of BMAL1 control circadian output functions in mouse liver. PLoS Biol. 9, e1000595 (2011).
Koike, N. et al. Transcriptional architecture and chromatin landscape of the core circadian clock in mammals. Science 338, 349–354 (2012).
Duong, H.A., Robles, M.S., Knutti, D. & Weitz, C.J. A molecular mechanism for circadian clock negative feedback. Science 332, 1436–1439 (2011).
Bannister, A.J. & Kouzarides, T. Regulation of chromatin by histone modifications. Cell Res. 21, 381–395 (2011).
Eberharter, A. & Becker, P.B. Histone acetylation: a switch between repressive and permissive chromatin. EMBO Rep. 3, 224–229 (2002).
Nielsen, S.J. et al. Rb targets histone H3 methylation and HP1 to promoters. Nature 412, 561–565 (2001).
Czermin, B. et al. Physical and functional association of SU(VAR)3–9 and HDAC1 in Drosophila. EMBO Rep. 2, 915–919 (2001).
Ripperger, J.A. & Schibler, U. Rhythmic CLOCK–BMAL1 binding to multiple E-box motifs drives circadian Dbp transcription and chromatin transitions. Nat. Genet. 38, 369–374 (2006).
Kwon, S.H. & Workman, J.L. The changing faces of HP1: from heterochromatin formation and gene silencing to euchromatic gene expression: HP1 acts as a positive regulator of transcription. Bioessays 33, 280–289 (2011).
Hiragami, K. & Festenstein, R. Heterochromatin protein 1: a pervasive controlling influence. Cell. Mol. Life Sci. 62, 2711–2726 (2005).
Shinkai, Y. & Tachibana, M. H3K9 methyltransferase G9a and the related molecule GLP. Genes Dev. 25, 781–788 (2011).
Robles, M.S., Boyault, C., Knutti, D., Padmanabhan, K. & Weitz, C.J. Identification of RACK1 and protein kinase Cα as integral components of the mammalian circadian clock. Science 327, 463–466 (2010).
Zhao, W.N. et al. CIPC is a mammalian circadian clock protein without invertebrate homologues. Nat. Cell Biol. 9, 268–275 (2007).
Peters, A.H. et al. Partitioning and plasticity of repressive histone methylation states in mammalian chromatin. Mol. Cell 12, 1577–1589 (2003).
Rea, S. et al. Regulation of chromatin structure by site-specific histone H3 methyltransferases. Nature 406, 593–599 (2000).
Vaute, O., Nicolas, E., Vandel, L. & Trouche, D. Functional and physical interaction between the histone methyl transferase Suv39h1 and histone deacetylases. Nucleic Acids Res. 30, 475–481 (2002).
Etchegaray, J.P., Lee, C., Wade, P.A. & Reppert, S.M. Rhythmic histone acetylation underlies transcription in the mammalian circadian clock. Nature 421, 177–182 (2003).
Taylor, P. & Hardin, P.E. Rhythmic E-box binding by CLK-CYC controls daily cycles in per and tim transcription and chromatin modifications. Mol. Cell. Biol. 28, 4642–4652 (2008).
Belden, W.J., Loros, J.J. & Dunlap, J.C. Execution of the circadian negative feedback loop in Neurospora requires the ATP-dependent chromatin-remodeling enzyme CLOCKSWITCH. Mol. Cell 25, 587–600 (2007).
Kwon, S.H. & Workman, J.L. HP1c casts light on dark matter. Cell Cycle 10, 625–630 (2011).
Saint-André, V., Batsche, E., Rachez, C. & Muchardt, C. Histone H3 lysine 9 trimethylation and HP1γ favor inclusion of alternative exons. Nat. Struct. Mol. Biol. 18, 337–344 (2011).
Vakoc, C.R., Mandat, S.A., Olenchock, B.A. & Blobel, G.A. Histone H3 lysine 9 methylation and HP1γ are associated with transcription elongation through mammalian chromatin. Mol. Cell 19, 381–391 (2005).
du Chéné, I. et al. Suv39h1 and HP1γ are responsible for chromatin-mediated HIV-1 transcriptional silencing and post-integration latency. EMBO J. 26, 424–435 (2007).
Choi, J.D., Park, M.A. & Lee, J.S. Suppression and recovery of BRCA1-mediated transcription by HP1γ via modulation of promoter occupancy. Nucleic Acids Res. 40, 11321–11338 (2012).
Storch, K.F. et al. Intrinsic circadian clock of the mammalian retina: importance for retinal processing of visual information. Cell 130, 730–741 (2007).
Chan, K.M. & Zhang, Z. Leucine-rich repeat and WD repeat-containing protein 1 is recruited to pericentric heterochromatin by trimethylated lysine 9 of histone H3 and maintains heterochromatin silencing. J. Biol. Chem. 287, 15024–15033 (2012).
Wolf, D. et al. Primer binding site-dependent restriction of murine leukemia virus requires HP1 binding by TRIM28. J. Virol. 82, 4675–4679 (2008).
Cai, W. et al. Expression levels of estrogen receptor β are modulated by components of the molecular clock. Mol. Cell. Biol. 28, 784–793 (2008).
Nishii, K. et al. Rhythmic post-transcriptional regulation of the circadian clock protein mPER2 in mammalian cells: a real-time analysis. Neurosci. Lett. 401, 44–48 (2006).
Lee, C. et al. Posttranslational mechanisms regulate the mammalian circadian clock. Cell 107, 855–867 (2001).
Acknowledgements
We thank T. Jenuwein for valuable discussion. This work was supported by a grant from the G. Harold & Leila Y. Mathers Charitable Foundation (C.J.W.) and a US National Institutes of Health Training grant T32NS007484 (H.A.D.).
Author information
Authors and Affiliations
Contributions
H.A.D. and C.J.W. designed the experiments and analyzed the data, H.A.D. performed the experiments, and C.J.W. oversaw the project.
Corresponding author
Ethics declarations
Competing interests
The authors declare no competing financial interests.
Integrated supplementary information
Supplementary Figure 1 HP1γ–Suv39h histone methyltransferase is a constituent of a PER complex in vivo: additional coimmunoprecipitations.
(a) Nuclear extract of mouse liver (input) obtained at CT18 and immunoprecipitates (IP) from the extract (antibodies indicated at top) were probed with antibodies to the proteins indicated at right. The abundant nuclear protein U2AF65 served as negative control and IgG-LC (light chain) served as positive control for immunoprecipitation. (b) Nuclear extract of mouse lung (input) obtained at CT18 and immunoprecipitates (IP) from the extract (antibodies indicated at top) were probed with antibodies to the proteins indicated at right. Abundant nuclear protein U2AF65 served as negative control.
Supplementary Figure 2 Suv39h2 histone methyltransferase catalytic subunit is important for circadian-feedback transcriptional repression and circadian-clock function.
(a) Western blot showing the effect of point-mutant control Suv39h2 siRNA or Suv39h2 siRNA on the steady-state abundance of endogenous Suv39h2 in mouse fibroblasts. Non-specific band served as loading control. (b) Depletion of Suv39h2 from mouse fibroblasts causes a specific increase in transcription of Clock-Bmal1 circadian target genes. Quantitative RT-PCR assays showing the steady-state abundance of the indicated pre-mRNAs in mouse fibroblasts after introduction of point-mutant control siRNA (black) or after depletion of Suv39h2 by Suv39h2 siRNA (gray; data normalized to control condition). Shown are mean +/− SEM of triplicate experiment; representative of 3 experiments (t-test, one-tailed). (c) Dual depletion of Suv39H1 and Suv39h2 from mouse fibroblasts. Quantitative RT-PCR assays showing the steady-state abundance of the indicated pre-mRNAs in mouse fibroblasts after introduction of point-mutant control siRNAs (black) or after depletion of Suv39h1 and Suv39h2 by Suv39h1 and Suv39h2 siRNAs (gray; data normalized to control condition; mean +/− SEM of triplicate experiments; N = 3 experiments; t-test, one-tailed). (d) Circadian oscillations of bioluminescence in synchronized reporter fibroblasts after delivery of point-mutant control siRNA (blue) or Suv39h2 siRNA (red). Traces from three independent cultures are shown for each. (e) Circadian periods of fibroblasts in (d) (mean +/− SEM; N = 3 for each condition; t-test, one-tailed).
Supplementary Figure 3 Suv39h1 and Suv39h2 are important for circadian-clock function: depletion by additional siRNAs.
(a) Western blot showing the effect of point-mutant control Suv39h1 siRNA or matched Suv39h1 siRNA on the steady-state abundance of endogenous Suv39h1 in cultured mouse fibroblasts. Non-specific band serves as loading control. (b) Circadian oscillations of bioluminescence in synchronized reporter fibroblasts after delivery of point-mutant control siRNA (blue) or Suv39h1 siRNA (red). Traces from three independent cultures are shown for each. (c) Circadian periods of fibroblasts in (b) (mean +/− SEM; N = 3 for each condition; t-test, one-tailed). (d-f) Analysis of Suv39h2, displayed as (a-c) above.
Supplementary Figure 4 HP1γ links chromatin-bound PER complex to Suv39h1: additional E boxes.
(a) ChIP assays showing HP1γ and Suv39h1 (as indicated) at a Per2 E-box site (left panels) or at the distal Per1 E-box site (right panels) in unsynchronized mouse fibroblasts after introduction of point-mutant control HP1γ siRNA (black) or after depletion of HP1γ by effective HP1γ siRNA (gray). ChIP values are normalized to signal from a parallel IgG control ChIP (dashed line); data are displayed as mean +/− SEM of a triplicate experiment and are representative of two independent experiments. (b) Diagram showing sites of Per1 and Per2 gene E-box ChIP primers relative to the transcription start sites (TSS, large arrowhead) of the two genes. Small arrowheads and open box, primer sites. (c) Up-regulation of HP1γ after depletion of Suv39h1 from mouse fibroblasts. Western blot showing the effect of point-mutant control Suv39h1 siRNA or matched Suv39h1 siRNA on the steady-state abundance of endogenous HP1γ in mouse fibroblasts. Non-specific band served as loading control. (d) Depletion of Suv39h1 has little or no effect on H3K9-Ac at Per1 and Per2 gene E-box sites. ChIP assays showing the H3K9 at Per1 (left) or Per2 (right) E-box sites in unsynchronized mouse fibroblasts after introduction of point-mutant control Suv39h1 siRNA (black) or after depletion of Suv39h1 by effective Suv39h1 siRNA (gray). ChIP values are normalized to the signal from a parallel IgG control ChIP (dashed line); data are displayed as mean +/− SEM of a triplicate experiment and are representative of two independent experiments.
Supplementary Figure 5 Distinct and ordered recruitment of HDAC1 and Suv39h1 to PER-encoding genes: additional E-box site.
ChIP assays showing temporal profiles of HDAC1, Suv39h1, PER1, and PER2 (as indicated at top left of each panel) at Per1 distal E-box site during the phase of circadian feedback transcriptional repression at 1-h time resolution. Shown are mean +/− SEM of triplicate experiments; representative of two independent ChIP assays from the 11 mice.
Supplementary Figure 6 Uncropped western blot images for Figure 1.
Red box marks the borders of the final cropped image for the indicated protein. Lanes: 1, Input; 2, IP: Control IgG; 3, IP: PER2 IgG. Numbers on right or left of image indicate positions of molecular weight markers (kD).
Supplementary Figure 7 Uncropped images for western blots in Figs. 3a and 6d.
Molecular weight markers (kD) are at right or left of each panel. Red box marks the borders of the final cropped image for the indicated protein. (a) For Fig. 3a. (b) For Fig. 6d. Lanes: 1, Input; 2, IP: Control IgG; 3, IP: PER2 IgG; 4, IP: PER2, followed by IP: Control IgG; 5, IP: PER2, followed by IP: HP1g IgG
Supplementary information
Supplementary Text and Figures
Supplementary Figures 1–7 (PDF 1326 kb)
Supplementary Table 1
RT-qPCR primers. (DOCX 17 kb)
Rights and permissions
About this article
Cite this article
Duong, H., Weitz, C. Temporal orchestration of repressive chromatin modifiers by circadian clock Period complexes. Nat Struct Mol Biol 21, 126–132 (2014). https://doi.org/10.1038/nsmb.2746
Received:
Accepted:
Published:
Issue Date:
DOI: https://doi.org/10.1038/nsmb.2746
This article is cited by
-
Mammalian PERIOD2 regulates H2A.Z incorporation in chromatin to orchestrate circadian negative feedback
Nature Structural & Molecular Biology (2022)
-
Stabilization of heterochromatin by CLOCK promotes stem cell rejuvenation and cartilage regeneration
Cell Research (2021)
-
Proteomic analysis of Drosophila CLOCK complexes identifies rhythmic interactions with SAGA and Tip60 complex component NIPPED-A
Scientific Reports (2020)
-
Molecular mechanisms and physiological importance of circadian rhythms
Nature Reviews Molecular Cell Biology (2020)
-
Circadian rhythms in the three-dimensional genome: implications of chromatin interactions for cyclic transcription
Clinical Epigenetics (2019)