Abstract
Huntington's disease (HD) is a progressive neurodegenerative disorder caused by a CAG trinucleotide repeat expansion in the huntingtin (HTT) gene, which encodes a polyglutamine tract in the HTT protein. We found that peroxisome proliferator-activated receptor delta (PPAR-δ) interacts with HTT and that mutant HTT represses PPAR-δ–mediated transactivation. Increased PPAR-δ transactivation ameliorated mitochondrial dysfunction and improved cell survival of neurons from mouse models of HD. Expression of dominant-negative PPAR-δ in the central nervous system of mice was sufficient to induce motor dysfunction, neurodegeneration, mitochondrial abnormalities and transcriptional alterations that recapitulated HD-like phenotypes. Expression of dominant-negative PPAR-δ specifically in the striatum of medium spiny neurons in mice yielded HD-like motor phenotypes, accompanied by striatal neuron loss. In mouse models of HD, pharmacologic activation of PPAR-δ using the agonist KD3010 improved motor function, reduced neurodegeneration and increased survival. PPAR-δ activation also reduced HTT-induced neurotoxicity in vitro and in medium spiny-like neurons generated from stem cells derived from individuals with HD, indicating that PPAR-δ activation may be beneficial in HD and related disorders.
This is a preview of subscription content, access via your institution
Access options
Subscribe to this journal
Receive 12 print issues and online access
$209.00 per year
only $17.42 per issue
Buy this article
- Purchase on Springer Link
- Instant access to full article PDF
Prices may be subject to local taxes which are calculated during checkout






Similar content being viewed by others
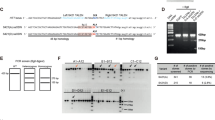
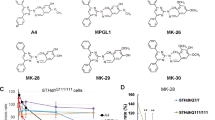
Accession codes
References
Berger, J. & Moller, D.E. The mechanisms of action of PPARs. Annu. Rev. Med. 53, 409–435 (2002).
Auboeuf, D. et al. Tissue distribution and quantification of the expression of mRNAs of peroxisome proliferator-activated receptors and liver X receptor-α in humans: no alteration in adipose tissue of obese and NIDDM patients. Diabetes 46, 1319–1327 (1997).
Kliewer, S.A. et al. Differential expression and activation of a family of murine peroxisome proliferator-activated receptors. Proc. Natl. Acad. Sci. USA 91, 7355–7359 (1994).
Luquet, S. et al. Peroxisome proliferator-activated receptor–δ controls muscle development and oxidative capability. FASEB J. 17, 2299–2301 (2003).
Schuler, M. et al. PGC1-α expression is controlled in skeletal muscles by PPAR-β, whose ablation results in fiber-type switching, obesity and type 2 diabetes. Cell Metab. 4, 407–414 (2006).
Wang, Y.X. et al. Regulation of muscle fiber type and running endurance by PPAR-δ. PLoS Biol. 2, e294 (2004).
Narkar, V.A. et al. AMPK and PPAR-δ agonists are exercise mimetics. Cell 134, 405–415 (2008).
Girroir, E.E. et al. Quantitative expression patterns of peroxisome proliferator-activated receptor-β/δ (PPAR-β/δ) protein in mice. Biochem. Biophys. Res. Commun. 371, 456–461 (2008).
Nance, M.A. Genetic testing of children at risk for Huntington’s disease. Neurology 49, 1048–1053 (1997).
Ross, C.A. et al. Huntington’s disease and dentatorubral-pallidoluysian atrophy: proteins, pathogenesis and pathology. Brain Pathol. 7, 1003–1016 (1997).
The Huntington’s Disease Collaborative Research Group. A novel gene containing a trinucleotide repeat that is expanded and unstable on Huntington’s disease chromosomes. Cell 72, 971–983 (1993).
La Spada, A.R. & Taylor, J.P. Repeat expansion disease: progress and puzzles in disease pathogenesis. Nat. Rev. Genet. 11, 247–258 (2010).
Beal, M.F. et al. Neurochemical and histologic characterization of striatal excitotoxic lesions produced by the mitochondrial toxin 3-nitropropionic acid. J. Neurosci. 13, 4181–4192 (1993).
Lin, M.T. & Beal, M.F. Mitochondrial dysfunction and oxidative stress in neurodegenerative diseases. Nature 443, 787–795 (2006).
Saudou, F., Finkbeiner, S., Devys, D. & Greenberg, M.E. Huntingtin acts in the nucleus to induce apoptosis but death does not correlate with the formation of intranuclear inclusions. Cell 95, 55–66 (1998).
Riley, B.E. & Orr, H.T. Polyglutamine neurodegenerative diseases and regulation of transcription: assembling the puzzle. Genes Dev. 20, 2183–2192 (2006).
Cui, L. et al. Transcriptional repression of PGC-1α by mutant huntingtin leads to mitochondrial dysfunction and neurodegeneration. Cell 127, 59–69 (2006).
Lin, J. et al. Defects in adaptive energy metabolism with CNS-linked hyperactivity in PGC-1α–null mice. Cell 119, 121–135 (2004).
Weydt, P. et al. Thermoregulatory and metabolic defects in Huntington’s disease transgenic mice implicate PGC-1α in Huntington’s disease neurodegeneration. Cell Metab. 4, 349–362 (2006).
Tsunemi, T. et al. PGC-1α rescues Huntington’s disease proteotoxicity by preventing oxidative stress and promoting TFEB function. Sci. Transl. Med. 4, 142ra97 (2012).
Gray, M. et al. Full-length human mutant huntingtin with a stable polyglutamine repeat can elicit progressive and selective neuropathogenesis in BACHD mice. J. Neurosci. 28, 6182–6195 (2008).
Chiang, M.C. et al. Modulation of energy deficiency in Huntington’s disease via activation of the peroxisome proliferator-activated receptor–γ. Hum. Mol. Genet. 19, 4043–4058 (2010).
Jin, Y.N., Hwang, W.Y., Jo, C. & Johnson, G.V. Metabolic state determines sensitivity to cellular stress in Huntington disease: normalization by activation of PPAR-γ. PLoS One 7, e30406 (2012).
Quintanilla, R.A., Jin, Y.N., Fuenzalida, K., Bronfman, M. & Johnson, G.V. Rosiglitazone treatment prevents mitochondrial dysfunction in mutant huntingtin–expressing cells: possible role of peroxisome proliferator-activated receptor–γ (PPAR-γ) in the pathogenesis of Huntington disease. J. Biol. Chem. 283, 25628–25637 (2008).
Bastie, C., Luquet, S., Holst, D., Jehl-Pietri, C. & Grimaldi, P.A. Alterations of peroxisome proliferator-activated receptor–δ activity affect fatty acid–controlled adipose differentiation. J. Biol. Chem. 275, 38768–38773 (2000).
Holst, D., Luquet, S., Kristiansen, K. & Grimaldi, P.A. Roles of peroxisome proliferator-activated receptors–δ and –γ in myoblast transdifferentiation. Exp. Cell Res. 288, 168–176 (2003).
Peters, J.M. et al. Growth, adipose, brain and skin alterations resulting from targeted disruption of the mouse peroxisome proliferator-activated receptor–β(δ). Mol. Cell. Biol. 20, 5119–5128 (2000).
Tronche, F. et al. Disruption of the glucocorticoid receptor gene in the nervous system results in reduced anxiety. Nat. Genet. 23, 99–103 (1999).
Guyenet, S.J. et al. A simple composite phenotype scoring system for evaluating mouse models of cerebellar ataxia. J. Vis. Exp. 39, e1787 (2010).
Shirendeb, U.P. et al. Mutant huntingtin’s interaction with mitochondrial protein Drp1 impairs mitochondrial biogenesis and causes defective axonal transport and synaptic degeneration in Huntington’s disease. Hum. Mol. Genet. 21, 406–420 (2012).
Song, W. et al. Mutant huntingtin binds the mitochondrial fission GTPase dynamin-related protein 1 and increases its enzymatic activity. Nat. Med. 17, 377–382 (2011).
Dang, M.T. et al. Disrupted motor learning and long-term synaptic plasticity in mice lacking NMDAR1 in the striatum. Proc. Natl. Acad. Sci. USA 103, 15254–15259 (2006).
Ferrante, R.J., Beal, M.F., Kowall, N.W., Richardson, E.P. Jr. & Martin, J.B. Sparing of acetylcholinesterase-containing striatal neurons in Huntington’s disease. Brain Res. 411, 162–166 (1987).
Reiner, A. et al. Striatal parvalbuminergic neurons are lost in Huntington’s disease: implications for dystonia. Mov. Disord. 28, 1691–1699 (2013).
Hodges, A. et al. Regional and cellular gene expression changes in human Huntington’s disease brain. Hum. Mol. Genet. 15, 965–977 (2006).
Willson, T.M., Brown, P.J., Sternbach, D.D. & Henke, B.R. The PPARs: from orphan receptors to drug discovery. J. Med. Chem. 43, 527–550 (2000).
Iwaisako, K. et al. Protection from liver fibrosis by a peroxisome proliferator-activated receptor–δ agonist. Proc. Natl. Acad. Sci. USA 109, E1369–E1376 (2012).
Schilling, G. et al. Intranuclear inclusions and neuritic aggregates in transgenic mice expressing a mutant N-terminal fragment of huntingtin. Hum. Mol. Genet. 8, 397–407 (1999).
Landis, S.C. et al. A call for transparent reporting to optimize the predictive value of preclinical research. Nature 490, 187–191 (2012).
Perrin, S. Preclinical research: make mouse studies work. Nature 507, 423–425 (2014).
Lu, M. et al. Brain PPAR-γ promotes obesity and is required for the insulin-sensitizing effect of thiazolidinediones. Nat. Med. 17, 618–622 (2011).
Chiang, M.C., Chern, Y. & Huang, R.N. PPAR-γ rescue of the mitochondrial dysfunction in Huntington’s disease. Neurobiol. Dis. 45, 322–328 (2012).
Jin, J. et al. Neuroprotective effects of PPAR-γ agonist rosiglitazone in N171-82Q mouse model of Huntington’s disease. J. Neurochem. 125, 410–419 (2013).
Zheng, B. et al. PGC-1α, a potential therapeutic target for early intervention in Parkinson’s disease. Sci. Transl. Med. 2, 52ra73 (2010).
Shin, J.H. et al. PARIS (ZNF746) repression of PGC-1α contributes to neurodegeneration in Parkinson’s disease. Cell 144, 689–702 (2011).
Evans, R.M. & Mangelsdorf, D.J. Nuclear receptors, RXR, and the big bang. Cell 157, 255–266 (2014).
Cramer, P.E. et al. ApoE-directed therapeutics rapidly clear β-amyloid and reverse deficits in AD mouse models. Science 335, 1503–1506 (2012).
Fitz, N.F., Cronican, A.A., Lefterov, I. & Koldamova, R. Comment on “ApoE-directed therapeutics rapidly clear β-amyloid and reverse deficits in AD mouse models.”. Science 340, 924 (2013).
Gines, S. et al. Specific progressive cAMP reduction implicates energy deficit in presymptomatic Huntington’s disease knock-in mice. Hum. Mol. Genet. 12, 497–508 (2003).
Young, J.E., Martinez, R.A. & La Spada, A.R. Nutrient deprivation induces neuronal autophagy and implicates reduced insulin signaling in neuroprotective autophagy activation. J. Biol. Chem. 284, 2363–2373 (2009).
Young, J.E. et al. Polyglutamine-expanded androgen receptor truncation fragments activate a Bax-dependent apoptotic cascade mediated by DP5 (Hrk). J. Neurosci. 29, 1987–1997 (2009).
Nithianantharajah, J., Barkus, C., Murphy, M. & Hannan, A.J. Gene-environment interactions modulating cognitive function and molecular correlates of synaptic plasticity in Huntington’s disease transgenic mice. Neurobiol. Dis. 29, 490–504 (2008).
Sopher, B.L. et al. Androgen receptor YAC–transgenic mice recapitulate SBMA motor neuronopathy and implicate VEGF164 in the motor neuron degeneration. Neuron 41, 687–699 (2004).
Garden, G.A. et al. Polyglutamine-expanded ataxin-7 promotes non–cell-autonomous Purkinje cell degeneration and displays proteolytic cleavage in ataxic transgenic mice. J. Neurosci. 22, 4897–4905 (2002).
La Spada, A.R. et al. Polyglutamine-expanded ataxin-7 antagonizes CRX function and induces cone-rod dystrophy in a mouse model of SCA7. Neuron 31, 913–927 (2001).
Janssen, A.J. et al. Spectrophotometric assay for complex I of the respiratory chain in tissue samples and cultured fibroblasts. Clin. Chem. 53, 729–734 (2007).
Aubry, L. et al. Striatal progenitors derived from human ES cells mature into DARPP32 neurons in vitro and in quinolinic acid–lesioned rats. Proc. Natl. Acad. Sci. USA 105, 16707–16712 (2008).
HD iPSC Consortium. Induced pluripotent stem cells from patients with Huntington’s disease show CAG-repeat expansion–associated phenotypes. Cell Stem Cell 11, 264–278 (2012).
Acknowledgements
We are grateful to S. Luquet (Université Paris Diderot) for the gift of the CAGGS-floxed-STOP-Ppard expression construct. ST-Hdh cells were a kind gift from M. MacDonald20,49 (Massachusetts General Hospital). BAC-HD97 mice21 were originally obtained from X.W. Yang (David Geffen School of Medicine at UCLA). This work was supported by funding from the Hereditary Disease Foundation, the Cure Huntington's Disease Initiative and grants from the US National Institutes of Health (R01 NS065874 (A.R.L.S.), R01 AG033082 (A.R.L.S.), National Research Service Award F32 NS081964 (A.S.D.) and P01 HL110873 (E.R.L.)).
Author information
Authors and Affiliations
Contributions
A.R.L.S. provided the conceptual framework for the study. A.S.D., V.V.P., T.T., B.L.S., E.R.L., G.W.Y., C.A.R., G.K.M., A.B.P., E.M. and A.R.L.S. designed the experiments. A.S.D., V.V.P., P.P.L., H.C.M., S.K.G.-H., N.L., K.R.S., A.B., M.-J.M.T., A.L.F., M.A., N.A., S.S.A., T.G., B.L.S., E.R.L., G.W.Y., E.M., G.K.M., A.B.P. and A.R.L.S. performed the experiments. A.S.D. and A.R.L.S. wrote the manuscript.
Corresponding author
Ethics declarations
Competing interests
The authors declare no competing financial interests.
Supplementary information
Supplementary Text and Figures
Supplementary Tables 1–2 and Supplementary Figures 1–13 (PDF 8222 kb)
Rights and permissions
About this article
Cite this article
Dickey, A., Pineda, V., Tsunemi, T. et al. PPAR-δ is repressed in Huntington's disease, is required for normal neuronal function and can be targeted therapeutically. Nat Med 22, 37–45 (2016). https://doi.org/10.1038/nm.4003
Received:
Accepted:
Published:
Issue Date:
DOI: https://doi.org/10.1038/nm.4003
This article is cited by
-
Abnormal molecular signatures of inflammation, energy metabolism, and vesicle biology in human Huntington disease peripheral tissues
Genome Biology (2022)
-
The psychiatric risk gene BRD1 modulates mitochondrial bioenergetics by transcriptional regulation
Translational Psychiatry (2022)
-
Recent Insights on the Role of PPAR-β/δ in Neuroinflammation and Neurodegeneration, and Its Potential Target for Therapy
NeuroMolecular Medicine (2021)
-
Erucic acid, a nutritional PPARδ-ligand may influence Huntington’s disease pathogenesis
Metabolic Brain Disease (2020)
-
Twist1 Plays an Anti-apoptotic Role in Mutant Huntingtin Expression Striatal Progenitor Cells
Molecular Neurobiology (2020)