Abstract
Metabolism of dietary glycans is pivotal in shaping the human gut microbiota. However, the mechanisms that promote competition for glycans among gut commensals remain unclear. Roseburia intestinalis, an abundant butyrate-producing Firmicute, is a key degrader of the major dietary fibre xylan. Despite the association of this taxon to a healthy microbiota, insight is lacking into its glycan utilization machinery. Here, we investigate the apparatus that confers R. intestinalis growth on different xylans. R. intestinalis displays a large cell-attached modular xylanase that promotes multivalent and dynamic association to xylan via four xylan-binding modules. This xylanase operates in concert with an ATP-binding cassette transporter to mediate breakdown and selective internalization of xylan fragments. The transport protein of R. intestinalis prefers oligomers of 4–5 xylosyl units, whereas the counterpart from a model xylan-degrading Bacteroides commensal targets larger ligands. Although R. intestinalis and the Bacteroides competitor co-grew in a mixed culture on xylan, R. intestinalis dominated on the preferred transport substrate xylotetraose. These findings highlight the differentiation of capture and transport preferences as a possible strategy to facilitate co-growth on abundant dietary fibres and may offer a unique route to manipulate the microbiota based on glycan transport preferences in therapeutic interventions to boost distinct taxa.
This is a preview of subscription content, access via your institution
Access options
Access Nature and 54 other Nature Portfolio journals
Get Nature+, our best-value online-access subscription
$29.99 / 30 days
cancel any time
Subscribe to this journal
Receive 12 digital issues and online access to articles
$119.00 per year
only $9.92 per issue
Buy this article
- Purchase on Springer Link
- Instant access to full article PDF
Prices may be subject to local taxes which are calculated during checkout





Similar content being viewed by others
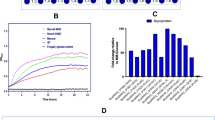
References
Nicholson, J. K. et al. Host–gut microbiota metabolic interactions. Science 336, 1262–1267 (2012).
Sonnenburg, J. L. & Bäckhed, F. Diet–microbiota interactions as moderators of human metabolism. Nature 535, 56–64 (2016).
Marchesi, J. R. et al. The gut microbiota and host health: a new clinical frontier. Gut 65, 330–339 (2016).
David, L. A. et al. Diet rapidly and reproducibly alters the human gut microbiome. Nature 505, 559–563 (2013).
Desai, M. S. et al. A dietary fiber-deprived gut microbiota degrades the colonic mucus barrier and enhances pathogen susceptibility. Cell 167, 1339–1353 (2016).
Cockburn, D. W. & Koropatkin, N. M. Polysaccharide degradation by the intestinal microbiota and its influence on human health and disease. J. Mol. Biol. 428, 3230–3252 (2016).
Xu, S. et al. Butyrate induces apoptosis by activating PDC and inhibiting complex I through SIRT3 inactivation. Signal Transduct. Target. Ther. 2, e16035 (2017).
Donohoe, D. R. et al. The Warburg effect dictates the mechanism of butyrate-mediated histone acetylation and cell proliferation. Mol. Cell 48, 612–626 (2012).
Furusawa, Y. et al. Commensal microbe-derived butyrate induces the differentiation of colonic regulatory T cells. Nature 504, 446–450 (2013).
Morrison, D. J. & Preston, T. Formation of short chain fatty acids by the gut microbiota and their impact on human metabolism. Gut Microbes 7, 189–200 (2016).
Takahashi, K. et al. Reduced abundance of butyrate-producing bacteria species in the fecal microbial community in Crohn’s disease. Digestion 93, 59–65 (2016).
Qin, J. et al. A metagenome-wide association study of gut microbiota in type 2 diabetes. Nature 490, 55–60 (2012).
Vrieze, A. et al. Transfer of intestinal microbiota from lean donors increases insulin sensitivity in individuals with metabolic syndrome. Gastroenterology 143, 913–916 (2012).
Duncan, S. H., Hold, G. L., Barcenilla, A., Stewart, C. S. & Flint, H. J. Roseburia intestinalis sp. nov., a novel saccharolytic, butyrate-producing bacterium from human faeces. Int. J. Syst. Evol. Microbiol. 52, 1615–1620 (2002).
Louis, P. & Flint, H. J. Diversity, metabolism and microbial ecology of butyrate-producing bacteria from the human large intestine. FEMS Microbiol. Lett. 294, 1–8 (2009).
Van den Abbeele, P. et al. Butyrate-producing Clostridium cluster XIVa species specifically colonize mucins in an in vitro gut model. ISME J. 7, 949–961 (2013).
El Kaoutari, A., Armougom, F., Gordon, J. I., Raoult, D. & Henrissat, B. The abundance and variety of carbohydrate-active enzymes in the human gut microbiota. Nat. Rev. Microbiol. 11, 497–504 (2013).
Mirande, C. et al. Dietary fibre degradation and fermentation by two xylanolytic bacteria Bacteroides xylanisolvens XB1AT and Roseburia intestinalis XB6B4 from the human intestine. J. Appl. Microbiol. 109, 451–460 (2010).
Chassard, C., Goumy, V., Leclerc, M., Del’homme, C. & Bernalier-Donadille, A. Characterization of the xylan-degrading microbial community from human faeces. FEMS Microbiol. Ecol. 61, 121–131 (2007).
Sheridan, P. O. et al Polysaccharide utilization loci and nutritional specialization in a dominant group of butyrate-producing human colonic Firmicutes. Microb. Genomics 2, e000043 (2016).
Selvendran, R. R. Chemistry of plant cell walls and dietary fibre. Scand. J. Gastroenterol. 5521, 33–41 (1987).
Rogowski, A. et al. Glycan complexity dictates microbial resource allocation in the large intestine. Nat. Commun. 6, 7481 (2015).
Zhang, M. et al. Xylan utilization in human gut commensal bacteria is orchestrated by unique modular organization of polysaccharide-degrading enzymes. Proc. Natl Acad. Sci. USA 111, E3708–E3717 (2014).
Lombard, V., Golaconda Ramulu, H., Drula, E., Coutinho, P. M. & Henrissat, B. The carbohydrate-active enzymes database (CAZy) in 2013. Nucleic Acids Res. 42, D490–D495 (2014).
Kelly, G. et al. Structure of the cell-adhesion fragment of intimin from enteropathogenic Escherichia coli. Nat. Struct. Mol. Biol. 6, 313–318 (1999).
Ebbes, M. et al. Fold and function of the InlB B-repeat. J. Biol. Chem. 286, 15496–15506 (2011).
Karlsson, E. N. et al. The modular xylanase Xyn10A from Rhodothermus marinus is cell-attached, and its C-terminal domain has several putative homologues among cell-attached proteins within the phylum Bacteroidetes. FEMS Microbiol. Lett. 241, 233–242 (2004).
Ejby, M. et al. An ATP binding cassette transporter mediates the uptake of α-(1,6)-linked dietary oligosaccharides in Bifidobacterium and correlates with competitive growth on these substrates. J. Biol. Chem. 291, 20220–20231 (2016).
Ejby, M. et al. Structural basis for arabinoxylo-oligosaccharide capture by the probiotic Bifidobacterium animalis subsp. lactis Bl-04. Mol. Microbiol. 90, 1100–1112 (2013).
Honda, Y. & Kitaoka, M. A family 8 glycoside hydrolase from Bacillus halodurans C-125 (BH2105) is a reducing end xylose-releasing exo-oligoxylanase. J. Biol. Chem. 279, 55097–55103 (2004).
Sun, Z. et al. A novel three-component system-based regulatory model for D-xylose sensing and transport in Clostridium beijerinckii. Mol. Microbiol. 95, 576–589 (2015).
Duncan, S. H. et al. Wheat bran promotes enrichment within the human colonic microbiota of butyrate-producing bacteria that release ferulic acid. Environ. Microbiol. 18, 2214–2225 (2016).
Morrill, J. et al. The GH5 1,4-β-mannanase from Bifidobacterium animalis subsp. lactis Bl-04 possesses a low-affinity mannan-binding module and highlights the diversity of mannanolytic enzymes. BMC Biochem. 16, 26 (2015).
Cockburn, D. W. et al. Molecular details of a starch utilization pathway in the human gut symbiont Eubacterium rectale. Mol. Microbiol. 95, 209–230 (2015).
Ze, X. et al. Unique organization of extracellular amylases into amylosomes in the resistant starch-utilizing human colonic firmicutes bacterium Ruminococcus bromii. mBio 6, e01058-15 (2015).
De Paepe, K., Kerckhof, F.-M., Verspreet, J., Courtin, C. M. & Van de Wiele, T. Inter-individual differences determine the outcome of wheat bran colonization by the human gut microbiome. Environ. Microbiol. 19, 3251–3267 (2017).
Mahowald, M. A. et al. Characterizing a model human gut microbiota composed of members of its two dominant bacterial phyla. Proc. Natl Acad. Sci. USA 106, 5859–5864 (2009).
Biely, P. et al. Mode of action of acetylxylan esterases on acetyl glucuronoxylan and acetylated oligosaccharides generated by a GH10 endoxylanase. Biochim. Biophys. Acta 1830, 5075–5086 (2013).
Sørbotten, A., Horn, S. J., Eijsink, V. G. H. & Vårum, K. M. Degradation of chitosans with chitinase B from Serratia marcescens. FEBS J. 272, 538–549 (2005).
Cock, P. J. A. et al. Biopython: freely available Python tools for computational molecular biology and bioinformatics. Bioinformatics 25, 1422–1423 (2009).
Kim, D. et al. TopHat2: accurate alignment of transcriptomes in the presence of insertions, deletions and gene fusions. Genome Biol. 14, R36 (2013).
Langmead, B. & Salzberg, S. L. Fast gapped-read alignment with Bowtie 2. Nat. Methods 9, 357–359 (2012).
Anders, S., Pyl, P. T. & Huber, W. HTSeq-A Python framework to work with high-throughput sequencing data. Bioinformatics 31, 166–169 (2015).
Love, M. I., Huber, W. & Anders, S. Moderated estimation of fold change and dispersion for RNA-seq data with DESeq2. Genome Biol. 15, 550 (2014).
Dümmler, A., Lawrence, A.-M. & de Marco, A. Simplified screening for the detection of soluble fusion constructs expressed in E. coli using a modular set of vectors. Microb. Cell Fact. 4, 34 (2005).
Miller, G. L. Use of dinitrosalicylic acid reagent for determination of reducing sugar. Anal. Chem. 31, 426–428 (1959).
Roe, J. H. & Rice, E. W. A photometric method for the determination of free pentoses in animal tissue. J. Biol. Chem. 173, 507–512 (1948).
Deschatelets, L. & Yu, E. K. C. A simple pentose assay for biomass conversion studies. Appl. Microbiol. Biotechnol. 24, 379–385 (1986).
Dilokpimol, A. et al. Enzymatic synthesis of β-xylosyl-oligosaccharides by transxylosylation using two β-xylosidases of glycoside hydrolase family 3 from Aspergillus nidulans FGSC A4. Carbohydr. Res. 346, 421–429 (2011).
Govind, V., Young, K. & Maudsley, A. A. Proton NMR chemical shifts and coupling constants for brain metabolites. NMR Biomed. 13, 129–153 (2000).
Zhang, H., Neal, S. & Wishart, D. S. RefDB: a database of uniformly referenced protein chemical shifts. J. Biomol. NMR 25, 173–195 (2003).
Takeo, K. Affinity electrophoresis: principles and applications. Electrophoresis 5, 187–195 (1984).
Scott, K. P., Martin, J. C., Duncan, S. H. & Flint, H. J. Prebiotic stimulation of human colonic butyrate-producing bacteria and bifidobacteria, in vitro. FEMS Microbiol. Ecol. 87, 30–40 (2014).
Anand, S., Kaur, H. & Mande, S. S. Comparative in silico analysis of butyrate production pathways in gut commensals and pathogens. Front. Microbiol. 7, 1945 (2016).
Acknowledgements
We thank B. Henrissat, architecture et fonction des macromolécules biologiques, CNRS, Aix-Marseille University and the curator of CAZy, for his advice and discussions on the assignment of the novel CBMx and the esterase. We also thank M. Yadav, US Department of Agriculture, Agricultural Research Service, for the kind gift of cornbran xylan, and BioCHOS AS for providing the chitooligo (CHOS) sample. M. Due, T. Holm Madsen and C. Aaarup Christensen are thanked for their technical help in cloning recombinant proteins and the performance of binding experiments. We also wish to thank A. Schultz, H. Juel Martens and M. Hansen, PLEN, University of Copenhagen, for the use of the confocal laser scanning microscopy in the initial microscopy experiments. This project was funded by a Graduate School DTU Scholarship, Lyngby, Denmark. Additional fundings were from the Danish Research Council for Independent Research, Natural Sciences (DFF, FNU) by a Research Project 2 grant (grant ID: 4002-00297B), a BIONÆR project (grant number: 244259) and the Norwegian NMR Platform, NNP (F.L.A.) from the Research Council of Norway (226244). Carlsberg Foundation is acknowledged for an ITC instrument grant (2011-01-0598).
Author information
Authors and Affiliations
Contributions
Growth analysis was performed by M.L.L. Transcriptomic analysis was done by M.L.L., C.W. and D.A.E. Enzyme characterization was performed by M.L.L., M.E., S.S.P., F.L.A. and B.W. qPCR was done by M.L.L. and M.I.B. Microscopy was perfromed by M.L.L. and C.S. Experiments were designed by M.L.L. and M.A.H. The manuscript written by M.L.L. and M.A.H. with contributions from T.R.L., B.W. and F.L.A. Figures were prepared by M.L.L.
Corresponding author
Ethics declarations
Competing interests
The authors declare no competing interests.
Additional information
Publisher’s note: Springer Nature remains neutral with regard to jurisdictional claims in published maps and institutional affiliations.
Supplementary information
Supplementary Information
Supplementary Tables 2–14, Supplementary Figures 1– 9.
Supplementary Table 1
R. intestinalis L1–82 gene expression in response to xylose (X1) relative to glucose (Glc) obtained from RNA-seq analysis.
Rights and permissions
About this article
Cite this article
Leth, M.L., Ejby, M., Workman, C. et al. Differential bacterial capture and transport preferences facilitate co-growth on dietary xylan in the human gut. Nat Microbiol 3, 570–580 (2018). https://doi.org/10.1038/s41564-018-0132-8
Received:
Accepted:
Published:
Issue Date:
DOI: https://doi.org/10.1038/s41564-018-0132-8
This article is cited by
-
Engineering Saccharomyces cerevisiae for targeted hydrolysis and fermentation of glucuronoxylan through CRISPR/Cas9 genome editing
Microbial Cell Factories (2024)
-
Production of cello-oligosaccharides from corncob residue by degradation-synthesis reactions
Applied Microbiology and Biotechnology (2024)
-
Next-generation probiotics: the upcoming biotherapeutics
Molecular Biology Reports (2024)
-
Current models in bacterial hemicellulase-encoding gene regulation
Applied Microbiology and Biotechnology (2024)
-
Microbiome and tryptophan metabolomics analysis in adolescent depression: roles of the gut microbiota in the regulation of tryptophan-derived neurotransmitters and behaviors in human and mice
Microbiome (2023)