Abstract
Two-dimensional metal-organic frameworks (MOFs) have a wide variety of applications in molecular separation and other emerging technologies, including atomically thin electronics. However, due to the inherent fragility and strong interlayer interactions, high-quality MOF crystals of atomic thickness, especially isolated MOF crystal monolayers, have not been easy to prepare. Here, we report the self-condensation-assisted chemical vapour deposition growth of atomically thin MOF single-crystals, yielding monolayer single-crystals of poly[Fe(benzimidazole)2] up to 62 μm in grain sizes. By using transmission electron microscopy and high-resolution atomic force microscopy, high crystallinity and atomic-scale single-crystal structure are verified in the atomically MOF flakes. Moreover, integrating such MOFs with MoS2 to construct ultrathin van der Waals heterostructures is achieved by direct growth of atomically MOF single-crystals onto monolayer MoS2, and enables a highly selective ammonia sensing. These demonstrations signify the great potential of the method in facilitating the development of the fabrication and application of atomically thin MOF crystals.
Similar content being viewed by others
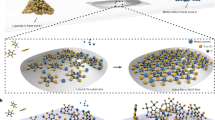
Introduction
Owing to the quantum confinement in two dimensions, single-layered crystals exhibit many exotic physical and chemical properties that differ from their bulk counterparts, thus triggering an explosion in the search for atomically thin materials1,2,3,4,5,6,7,8. Recently, two-dimensional (2D) metal-organic frameworks (MOFs) with inherent molecular pore/cavity structures that consist of metal ions connected by organic ligands have emerged as one increasingly compelling field due to diverse designable structures and tunable properties9,10,11,12,13. At the atomic thickness, 2D MOFs feature rapid mass transfer11, exceptional carrier transport13,14 and an extremely high proportion of exposed active sites15,16, and have easily identifiable atomic structures and bonding arrangements for an ideal model to search for precise structure-performance relationship17. Because of these unique characteristics, 2D MOFs have shown great potential as building blocks for significant technologies in applications such as molecular sensing18, gas separation11,19, catalysis15,16,17 and superconductor20. A key towards the fulfilment of these features and notable applications is to reliably prepare atomically thin MOF crystals in high quality. Great effort has thus been devoted to the development of their preparation methods, such as micromechanical exfoliation10, liquid exfoliation16,21, and wet-chemical method14,17. However, due to the inherent fragility and strong interlayer interactions in the bulk crystals, 2D MOFs obtained by the above methods are usually thick or have a few layers with small size and/or limited crystallinity. It is highly desired to reliably prepare high-quality and large MOF crystals with atomic thickness, especially the isolated monolayers.
Chemical vapour deposition (CVD), a typical bottom-up method, is considered to be a robust way to grow 2D materials with controllable thickness, scalable size and high crystal quality. A range of high-quality monolayer 2D materials, such as graphene22, h-BN6,23, and transition metal dichalcogenides (TMDs)24,25,26, have been prepared by the CVD method. Therefore, it is promising to prepare high-quality monolayer MOFs with large sizes through the CVD technique. Recently, a multistep CVD method has been demonstrated to achieve the growth of MOF thin films (such as ZIF-8)27, which marks notable progress in the CVD synthesis of MOFs. Nonetheless, several fundamental challenges remain in terms of CVD growth of atomically thin MOF single-crystals. In a typical CVD process for growing inorganic nanomaterials and graphene, atoms can diffuse rapidly over a long distance on the substrate surface at high growth temperatures (≥700 °C), resulting in the generation of large monolayer domains. While in the CVD growth of MOFs, a low growth temperature (typically below 300 °C) and strong interactions between ligand and substrate, as well as the large mass and volume of ligand molecules, together cause the ligand precursor molecules to have a short free path on the substrate surface. Dense nucleation and growth of small grains of tens of nanometres often ensue, resulting in thick polycrystalline films of MOFs. Thus far, the growth of large, single-crystal, atomically thin MOFs, especially for the monolayers, has yet to be realized using the CVD method.
Here, we demonstrate a self-condensation-assisted CVD (SCA-CVD) growth of atomically thin, single-crystal MOF, in which monolayer poly[Fe(benzimidazole)2] single-crystals were obtained with grain sizes up to 62 μm. The self-condensation of the precursor induced by a temperature gradient design during the CVD process is supposed to be critical in mediating the growth of atomically thin, large-sized MOF single crystals. Characterization by transmission electron microscopy (TEM) and high-resolution atomic force microscopy (HRAFM) have revealed the high crystallinity and atomic structures of single-crystals in the monolayer and few-layer MOF flakes. In addition, good compatibility of the SCA-CVD growth of MOF crystals has been shown and enabled atomically thin MOF single-crystals to grow directly onto monolayer MoS2 to create an ultrathin van der Waals (vdW) heterostructure of MOF/MoS2. Integration combining the precise gate effect of MOF crystal and the high sensitivity of monolayer MoS2 is testified in such heterostructure to engender highly selective ammonia sensing.
Results
SCA-CVD growth and characterization of atomically thin MOF single-crystals
The poly[Fe(benzimidazole)2], denoted as Fen(bim)2n, is a typical Van der Waals layered MOF. A structural illustration (Fig. 1a, b) of a single-crystal MOF of Fen(bim)2n shows a layered construction in which distorted tetrahedral Fe(II) centres are linked with bridging bis-monodentate benzimidazole ligands in the a–b plane10. We conducted the SCA-CVD growth of the atomically thin layer of Fen(bim)2n on SiO2/Si substrates using powdered ferrocene and benzimidazole as precursors in a two-zone tube furnace system (see the “Methods” section for details). Monolayer and few-layer Fen(bim)2n crystals were obtained and first identified under an optical microscope. As shown in Fig. 1c, d and Supplementary Fig. 1, a well-defined rectangle shape was clearly exhibited by the isolated flakes, which is well aligned with the coordination framework symmetry of Fen(bim)2n single-crystals. The exact thicknesses of the flakes were measured to be 1.1 and 3.5 nm (Fig. 1f, g), corresponding to monolayer and three-layer thicknesses, respectively. Statistics based on the AFM measurement showed that the ratio of the monolayer was ~50%. In addition, the Fen(bim)2n single-crystals grown by the SCA-CVD method were of considerable lateral dimensions, with lengths for the monolayer and the few-layer up to 62 and 105 μm, respectively. To the best of our knowledge, this was the first time monolayer MOF crystals were obtained with the CVD method, and the MOF crystal was also the largest monolayer single-crystal MOF obtained thus far. Furthermore, the SCA-CVD method allowed atomically thin single-crystal Fen(bim)2n to be grown on substrates, including KBr crystals, mica, sapphire and so on, where grown crystals showed a similar appearance to that grown on the SiO2/Si substrates (Supplementary Fig. 2). Also, atomically thin MOF crystals, including poly[Fe(5-methylbenzimidazole)2], poly[Fe(5-bromobenzimidazole)2], poly[Fe(5-chlorobenzimidazole)2] and poly[Zn(benzimidazole)2] were successfully prepared via SCA-CVD (Supplementary Figs. 3 and 4).
a Crystal structure of a Fen(bim)2n single crystal viewed down the c-axis (a–b plane), i.e., the lattice structure of a single layer of Fen(bim)2n, where the iron atoms are represented in orange, nitrogen atoms in blue, carbon atoms in grey and hydrogen atoms are omitted for clarity10. b Layered crystal structure of a Fen(bim)2n single crystal viewed along the b-axis10. c, d Optical images of a typical monolayer (c) and three-layered (d) Fen(bim)2n single crystal grown on a SiO2/Si substrate by SCA-CVD. e, f Corresponding AFM images of the Fen(bim)2n single crystal in (c) and (d). Scale bars, 10 μm in (c), 20 μm in (d) and 1 μm in (e, f).
For SCA-CVD growth of MOFs, ferrocene and various benzimidazole derivatives can be easily obtained in large quantities, facilitating the extension of prepared MOF types, and they are easy to sublimate. The benzimidazole derivatives also demonstrate a suitable melting point in which a temporary liquid environment can be offered. Figure 2 schematically shows SCA-CVD growth. Self-condensation of the precursor into liquid was found to be induced through a temperature gradient design (Fig. 2a) in the CVD process. As shown in Supplementary Fig. 5, obvious vapour-condensed liquid droplets of benzimidazole appeared on the surface of the growth substrate in the gradient zone and on the inner wall of the nearby quartz tube upon thermal sublimation of the precursor. Temperature monitoring (Supplementary Fig. 6) also showed that the substrate temperatures (~200 °C) during the growth stage were higher than the melting point (169–171 °C) of benzimidazole, indicating that the benzimidazole deposited on the substrate existed in liquid state at the growth stage. To further investigate the growth process, a control experiment was carried out. The self-condensed liquid droplets at different growth times were extracted from the same area of the growth substrate and analysed by gas chromatography‒mass spectrometry (GC‒MS) (Supplementary Fig. 7). A time-dependent evolution of components in the liquid droplets was revealed, in which the droplets gradually changed from pure benzimidazole into a mixture of benzimidazole and ferrocene. These experimental results depicted a probable process of SCA-CVD growth (Fig. 2b). First, benzimidazole precursor was sublimated into vapour, diffused to the substrate, and then condensed into liquid upon a negative temperature gradient. The vapourized ferrocene subsequently dissolved and diffused in the benzimidazole droplets, accompanied by coordination reactions with benzimidazole molecules. Lastly, the Fen(bim)2n nucleated and grew into crystals on the substrate. It should be noted that the involved liquid droplets in the SCA-CVD growth are formed upon vapourization to condensation, and thus they are of high purity and can be fully removed from the growing crystals by a simple vacuum treatment due to the volatility of the precursors without leaving impurities, which is completely different from ordinary solvents and in situ formed liquids.
a Schematics of the set-up for SCA-CVD growth. b The proposed process of SCA-CVD growth involves liquid droplet formation due to the self-condensation of benzimidazole precursor vapour, dissolution and diffusion of the vapourized ferrocene molecules in the liquid droplets, formation of atomically thin single crystals of Fen(bim)2n.
To examine the crystal quality and structure of the SCA-CVD-grown Fen(bim)2n flakes, TEM, selective area electron diffraction (SAED), and HRAFM characterizations were performed. Fen(bim)2n flakes grown on KBr crystals were employed for TEM observation, as the excellent water solubility of KBr crystals allows Fen(bim)2n flakes to be gently transferred to TEM grids in water, thus preserving intrinsic properties of the transferred flakes. As shown in Fig. 3a, b, monolayer and few-layer Fen(bim)2n flakes exhibit typical rectangular shapes with clean and uniform surfaces, which are consistent with the observations obtained by the optical microscope. Collected along the [001] axis, SAED patterns of these flakes (inset of Fig. 3a, b) show clear Bragg diffraction signals and only one set of quasi-fourfold symmetry diffraction spots, which is in good agreement with the simulated electron diffraction pattern (Supplementary Fig. 8), suggesting that both the single-layer and few-layer Fen(bim)2n flakes are single-crystals with high crystallinity. An individual Fen(bim)2n flake in a rectangle shape has been further confirmed as a single crystal by collecting SAED patterns at different positions throughout the entire flake (Supplementary Figs. 9–11). A regular periodic lattice structure is displayed in the few-layer Fen(bim)2n flake, as shown in the cryogenic TEM image (Fig. 3d). The lattice spacing was measured to be ~2.9 Å, close to the d220 of the Fen(bim)2n crystal, which corresponds to (002) plane of the Fen(bim)2n crystal. Almost no point defects and voids were initially observed within the entire field of view, further confirming high crystallinity of the Fen(bim)2n flake. As monolayer MOFs suffer visible and rapid disordering under electron beam irradiation, which is common for 2D MOF crystal characterization with high-resolution TEM, we examined the atomic structure of monolayer Fen(bim)2n with HRAFM. As shown in Fig. 3c and Supplementary Fig. 12, rectangular Fen(bim)2n units interconnecting into a periodically arranged network with an internal angle of about 90° are clearly observed in the monolayer. The lattice constants of a and b were measured to be 8.3 and 8.4 Å, respectively, which matched well with the crystallographic data, suggesting good quality of the grown monolayer Fen(bim)2n. The elemental information and chemical bonding of Fen(bim)2n were also confirmed by scanning transmission electron microscopy (STEM) energy-dispersive X-ray spectroscopy (EDS) mapping images (Supplementary Fig. 13), X-ray photoelectron spectroscopy (XPS, Supplementary Fig. 14) and Fourier transform infra-red (FT-IR, Supplementary Fig. 15).
a Low-magnification TEM image of a typical monolayer Fen(bim)2n single crystal. The inset shows the corresponding SAED pattern in (a). b Low-magnification TEM image of a typical few-layer Fen(bim)2n single crystal, with its SAED pattern shown in the inset. c HRAFM image of a typical monolayer Fen(bim)2n single crystal. d Cryogenic TEM image of a typical few-layer Fen(bim)2n single crystal. The inset shows the higher-magnification image, corresponding to the (002) plane of the Fen(bim)2n crystal. The cryogenic TEM was conducted under a low dose of 17.56 e/Å2/s. Scale bars, 2 μm in (a), 5 μm in (b), 2 nm in (c), 10 nm in (d) and 1 nm in inset of (d).
Mechanism of SCA-CVD growth of single-crystal 2D MOFs
Compared with previous studies, the SCA-CVD method demonstrates the following advantages: it has achieved the growth of large-sized single-layer MOFs and a relatively high single-layer ratio by simple one-step operations, and the grown 2D MOFs single crystals are clean and of good crystallinity. The involvement of the self-condensation liquid in the CVD process is supposed to play a vital role in enabling the growth of the atomically thin large-sized Fen(bim)2n single-crystals. During the traditional CVD process for 2D MOF crystals growing, the coordination of precursors in the gas phase is difficult without catalysts; the low growth temperature typically leads to irreversible crystal growth, resulting in products with poor crystallinity, and kinetic limitations caused by small diffusion rates and radii of the localized ligand precursors also tend to generate small grains. In the SCA-CVD method for MOF growth, a pure temporary liquid environment is introduced into the growth system through the self-condensation of the precursor. This liquid environment serves as a buffer layer to reduce disturbance inside and outside the liquid on the crystal nucleation and growth and allows precursor molecules to be uniformly and stably distributed within the liquid. An increased diffusion rate and radius of the precursor molecules compared with that on the gas–solid interface can be achieved in the liquid to lessen the kinetic limitations of large grain growth along the lateral direction. In addition, similar to most 2D materials, the atoms in a Fen(bim)2n layer interact through strong chemical bonds, while the interactions between layers are weak van der Waals force10. Previous density functional theory (DFT) studies have shown that the layer−layer interactions in most van der Waals materials are around 0.03–0.2 eV per atom, while the strength of typical chemical bonds is between 2 and 8 eV per atom28. The strength of intralayer interaction is 1–2 orders of magnitude higher than that of interlayer interaction. Because of the stronger intralayer binding, attaching atoms to an edge of a Fen(bim)2n layer to facilitate the in-plane extension is much easier than adsorbing atoms on the surface of a Fen(bim)2n layer for the growth of multilayers. More specifically, the nucleation energy for a new layer is the maximum of \(\alpha \sqrt{A}-\beta A\), where \(A\) is the nucleus size, and the first and second terms represent the positive and negative contributions from the edge and bulk of the nucleus, respectively29. In comparison, the nucleation energy for the growth of an edge (in-plane extension) is the energy of new dangling bonds when forming a unitcell of Fen(bim)2n on the edge30. Under a near equilibrium growth condition (low β), the critical nucleation size for a new layer will be much larger than a unitcell of Fen(bim)2n, and more dangling bonds involves in the formation of a new layer, which results in a higher nucleation energy for a new layer. Therefore, selective in-plane growth of Fen(bim)2n is favourable in an equilibrium reversible thermodynamic process, as the case in CVD growth of most 2D Van der Waals crystals. Notably, the liquid nature allows dynamic bonding between the metal and ligand nodes of MOFs, in which the metal−ligand bonds formed, broke and reformed to correct disorder or premature structure termination, which is crucial in forming a crystalline and ordered structure31. In addition, as the separate nuclei in the liquid grow and approach each other, due to the preference of the free energy of the system to decrease, interface elimination processes prefer to occur to reduce the total area of the grain boundaries32. It energetically favoured the formation of large-sized, single-crystalline MOF crystals in the liquid environment. These ultimately afford the growth of large single-crystal 2D MOFs.
Selective ammonia sensing of ultrathin vdW heterostructure of Fen(bim)2n/MoS2
The demonstrations of high-quality growth of atomically thin Fen(bim)2n flakes make it desirable to explore their integration into devices. Ultrathin vdW heterostructures constitute a simple but powerful platform for studying fundamental physics and creating functional devices in the 2D limit, in which combining or extending properties are accessible through the synergy of their constituent materials33. Since the SCA-CVD method is general to a variety of substrates, we fabricated a 2D vdW heterostructure composed of atomically thin Fen(bim)2n single-crystal directly grown onto MoS2 monolayers via the SCA-CVD method in which the transfer processes that often cause quality damage and contamination are not involved. (Fig. 4 and Supplementary Fig. 16; see the “Methods” section for details). No obvious interaction between Fen(bim)2n and MoS2 was observed in the heterostructure (Supplementary Fig. 17). Monolayer MoS2 is characterized by a high sensitivity response due to its high surface-to-volume ratio and excellent semiconducting properties. To evaluate such vdW heterostructures, gas-sensing measurements were performed. The large size of SCA-CVD grown Fen(bim)2n single crystals enables the direct construction of electrodes for the heterostructure sensors using a hollow mask method without the use of electron beam lithography technology (see the “Methods” section). Figure 4c shows a typical sensing response (S) of a Fen(bim)2n/MoS2 sensor (Supplementary Fig. 18) to varied concentrations of NH3 gas, where S is defined as (RSensor−R0)/R0, R0 and RSensor are the resistance of the sensor before and after gas introduction. The sensor responded almost immediately to NH3 exposure, given that the response is limited by the opening speed of the solenoid valve and the gas mixing speed in the sensing chamber. Clear electrical resistance decreases were observed in the sensors upon NH3 exposure, even at sub-ppm levels (500 ppb). The sensitivity upon NH3 exposure is found to be comparable to that of independent 2D MoS2 sensors34, which suggests the good sensitivity of the Fen(bim)2n/MoS2. In addition, good linear sensor sensitivity can be achieved when the NH3 concentration ranges from 1 to 100 ppm (Fig. 4d), indicating a feasible determination of gas concentration.
a Optical image of an ultrathin vdW heterostructure of Fen(bim)2n/MoS2. b Schematic illustration of a device based on the vdW heterostructure of Fen(bim)2n/MoS2, in which the periodic network of Fen(bim)2n represented by the dark grey grid is superimposed on a monolayer MoS2 denoted as atomic structure. c Real-time sensing behaviour of a Fen(bim)2n/MoS2 sensor upon consequent NH3 exposures at various concentrations. The inset shows an optical image of the Fen(bim)2n/MoS2 sensor. ∆R is the change in sensor resistance, defined as RSensor−R0. Scale bar, 20 μm. d Plot of resistance change (black solid squares) of Fen(bim)2n/MoS2 sensor as a function of NH3 concentration. The red line indicates the fitted line. e Sensing response of a monolayer MoS2 (black line) and a Fen(bim)2n/MoS2 (red line) when exposed to NH3 gas, tert-butylamine (TBA) vapour, tert-pentylamine (TPA) vapour, isopropylamine (IPA) vapour and 2-butylamine (2-BA) vapour of the same concentration (20 ppm). The blue arrow represents the injection of analytes. f Normalized sensing response of the monolayer MoS2 and the Fen(bim)2n/MoS2 heterostructure in (e). c–f are provided as a Source Data file.
Independent monolayer MoS2 is highly sensitive to environmental active stimuli, as their atoms are almost completely exposed; however, it is theoretically impossible to distinguish interfering substances with similar chemical properties by monolayer MoS2. Amine gases/vapours such as NH3, tert-butylamine (TBA), tert-pentylamine (TPA), isopropylamine (IPA) and 2-butylamine (2-BA) are electron donors; When expose them to a monolayer MoS2, the absorbed molecules on the surface of MoS2 would shift the Fermi level to the conduction band, leading to electrical resistance decreases of MoS233. As shown in Fig. 4e, obvious electrical resistance decreases were all observed in a monolayer MoS2 sensor to exposure of NH3, TBA, TPA, IPA and 2-BA, respectively, with S of −5.45% for NH3, −1.82% for TBA, −2.63% for TPA, −4.21% for IPA and −2.74% for 2-BA (at the same concentration). However, when exposing the above analytes to sensors fabricated with the Fen(bim)2n/MoS2 heterostructure, a rapid drop in device resistance only occurs as gaseous NH3 is injected, and almost no change in the resistance is observed with an injection of other amine vapours. The S of the Fen(bim)2n/MoS2 sensor for the same concentrations of NH3, TBA, TPA, IPA and 2-BA are about −4.14%, −0.11%, −0.08%, −0.10% and −0.09%, respectively. Calculating selectivity coefficient of the NH3 (\(D_{{\rm NH}_{3}}\)) that is defined as the ratio of responses of NH3 and another interfered gas/vapour, that is \(D_{{\rm NH}_{3}}\) = \(S_{{\rm NH}_{3}}\)/Si, there is at least one order of magnitude increase in \(D_{{\rm NH}_{3}}\) for the Fen(bim)2n/MoS2 (Fig. 4f), where \(D_{{\rm NH}_{3}}\) was 2.99 to TBA, 2.07 to TPA, 1.29 to IPA and 1.99 to 2-BA for the monolayer MoS2 sensor, and 36.95 to TBA, 50.19 to TPA, 40.18 to IPA and 43.32 to 2-BA for the Fen(bim)2n/MoS2 sensor. This proves a clearly enhanced NH3 selective response of the Fen(bim)2n/MoS2 heterostructure among amine vapours/gases. In addition, the NH3 selectivity of the Fen(bim)2n/MoS2 sensor has good reproducibility (Supplementary Fig. 19).
The good sensitivity and selective ammonia response of the ultrathin vdW heterostructure of Fen(bim)2n/MoS2 (Supplementary Table 1) reflect the integration of the high sensitivity of monolayer MoS2 and the precise gate effect of MOF crystals. The MOF crystal of Fen(bim)2n is known to be a microporous crystalline material with inherent size exclusion characteristics. According to the crystallographic data, the theoretical aperture size of Fen(bim)2n unit is estimated to be about 0.21 nm (Supplementary Fig. 20). Considering the structure flexibility of MOF owing to the aromatic rings flip-flop, the effective pore size should be slightly larger than the theoretical aperture size11,35. Organic amine vapours, including TBA, TPA, IPA and 2-BA, all have kinetic diameters far exceeding the effective aperture size of Fen(bim)2n. As expected, due to the size exclusion effect by the small aperture size of Fen(bim)2n, the gate effect of the Fen(bim)2n layer prevents these organic amine vapours from accessing the surface of MoS2, causing almost no gas sensing responses. While NH3 molecules with a kinetic diameter of ~2.6 Å, which is comparable to the effective pore aperture of Fen(bim)2n, can easily pass through the Fen(bim)2n network and reach the MoS2 surface, resulting in gas responses. Notably, when epitaxially grown onto monolayer MoS2 forming a vdW heterostructure, the Fen(bim)2n crystals stacked compactly on the MoS2 with an atomically clean interface. In addition, the grown Fen(bim)2n crystals were intact single-crystals without grain boundary defects to generate undesired gas permeation pathways. These enable the MOF crystal to maximize its precise gate effect in the heterostructure, which is different from the case of previously reported MOF thin films. A strict nanochannel created by the Fen(bim)2n single-crystals is thus imposed on the monolayer MoS2, in which only molecules of a certain size are able to reach the MoS2. Besides, compared to the long nanopores in a MOF thin film, the Fen(bim)2n with atomic-level thickness presents ultra-short nanopores that allow the high sensitivity and fast response of MoS2 monolayers to be retained in the heterostructure, which is responsible for the measured good sensitivity of the Fen(bim)2n/MoS2 to NH3 exposure.
In conclusion, our results demonstrate the preparation of high-quality MOF single-crystals with atomic thicknesses using a SCA-CVD method, in which large isolated monolayer single-crystals of Fen(bim)2n in high crystallinity were achieved. By directly growing atomically thin MOF single-crystals on monolayer MoS2 via the SCA-CVD method, we fabricated ultrathin vdW heterostructures of Fen(bim)2n/MoS2, of which a highly selective ammonia response was shown to result from the synergy of MOF and MoS2. Our methodology inspires a synthetic pathway for creating high-quality atomically thin molecular frameworks that were previously inaccessible and can greatly promote their low-dimensional device integration and applications.
Methods
Growth of atomically thin Fen(bim)2n single crystals
The single-crystal Fen(bim)2n with atomic thickness was prepared by the SCA-CVD method at atmospheric pressure. Precursor powders, benzimidazole (Innochem, 99%, 0.15 mmol) and ferrocene (Adamas Beta, 99%, 0.15 mmol), were respectively loaded at two ends of a single-ended sealed quartz tube. Clean growth substrate, such as SiO2/Si and sapphire, was placed face up between the benzimidazole and ferrocene. The quartz tube containing precursors and growth substrate was then transferred into a two-zone furnace, with the benzimidazole and ferrocene, respectively, in the centres of the two heating zones and the growth substrate located in the temperature gradient zone. Before growth, the system was first degassed and then purged with ultra-purity nitrogen for 10 min at a flow rate of 200 sccm. The growth recipe was ramped from room temperature to 400 °C within 20 min for the heating zone (1, benzimidazole loading) while from room temperature to 150 °C for the heating zone (2, ferrocene loading) and sit 15 min at 400 and 150 °C. Ultra-purity nitrogen with 5 sccm was flowed in the whole growth process. After growth, the system was degassed to terminate the reaction and then opened the furnace for rapid cooling.
GC-MS analysis
The liquid droplet components during the SCA-CVD process were analysed by gas chromatography-mass spectrometry GC–MS (SHIMADZU-QP2010) with a packed column DB-5 MS. Droplets located at the same position of the substrates were collected by breaking off the heating programme at desired times. A mixture of benzimidazole and ferrocene with a molar ratio of 1:1 was used as a reference sample for the GC–-MS test. All samples for GC–MS testing utilized ethanol as a solvent.
Preparation of vdW 2D Fen(bim)2n/MoS2 heterostructures
We first grew monolayer MoS2 crystals on a SiO2/Si substrate by CVD using a method reported in ref. 24. The as-grown MoS2 monolayers were transferred to the two-zone furnace as substrates for the growth of Fen(bim)2n. The SCA-CVD growth of Fen(bim)2n was then conducted using the above similar recipes, in which atomically thin Fen(bim)2n crystals were directly grown on MoS2 monolayers. The vdW 2D Fen(bim)2n/MoS2 heterostructures were thus fabricated.
Device fabrication and measurements
The gas-sensing devices were fabricated through direct thermal evaporation deposition of 60 nm gold on top of the monolayer MoS2 flakes and Fen(bim)2n/MoS2 heterostructures with a metal shadow mask. Gas-sensing experiments were carried out at room temperature in a gas-sensing chamber, where the devices were placed on a chip-carrier with electrically connected gold wires led out by a vacuum terminal block. The gas sensing chamber was maintained at a pressure of 100 Pa and balanced with pure nitrogen at a flow rate of 100 sccm. Analytes we used for the test were NH3 gas, isopropylamine vapour, 2-butylamine vapour, tert-butylamine vapour and tert-pentylamine vapour. Mix them with ultra-pure nitrogen in proportion to achieve desirable concentrations, and then inject them into the gas-sensing chamber by mass flow controllers with a flow rate of 100 sccm. Adjust the flow rate of pure nitrogen to zero when introducing the analyte to maintain a total constant flow rate of 100 sccm. The current of the devices during the gas-sensing test was monitored in real-time at a constant bias voltage (−1.0 V) using the two-probe with an Agilent B2902A Source Meter.
Characterizations and simulation
Optical images were captured with a Nikon Eclipse LV 100D. AFM images were collected in tapping mode using a Bruker Dimension Icon Scanning Probe Microscope. HRAFM images were recorded on a Cypher VRS1250 in contact mode. The TEM experiments were carried out with a JEOL JEM-2100F operated at 200 kV. Cryogenic TEM image was obtained with a Thermo Scientific Themis 300 with a field-emission gun operating at 300 kV. Simulated SAED pattern was generated with SingleCrystal software.
References
Gao, L. et al. Face-to-face transfer of wafer-scale graphene films. Nature 505, 190–194 (2014).
Hou, L. et al. Synthesis of a monolayer fullerene network. Nature 606, 507–510 (2022).
Chen, T.-A. et al. Wafer-scale single-crystal hexagonal boron nitride monolayers on Cu (111). Nature 579, 219–223 (2020).
Liu, K. et al. On-water surface synthesis of crystalline, few-layer two-dimensional polymers assisted by surfactant monolayers. Nat. Chem. 11, 994–1000 (2019).
Zhao, X. et al. Engineering covalently bonded 2D layered materials by self-intercalation. Nature 581, 171–177 (2020).
Wang, M. et al. Exceptionally high charge mobility in phthalocyanine-based poly(benzimidazobenzophenanthroline)-ladder-type two-dimensional conjugated polymers. Nat. Mater. 22, 880–887 (2023).
Leng, K. et al. Molecularly thin two-dimensional hybrid perovskites with tunable optoelectronic properties due to reversible surface relaxation. Nat. Mater. 17, 908–914 (2018).
Zhang, X. et al. Delocalized electron effect on single metal sites in ultrathin conjugated microporous polymer nanosheets for boosting CO2 cycloaddition. Sci. Adv. 6, eaaz4824 (2020).
Chakraborty, G., Park, I.-H., Medishetty, R. & Vittal, J. J. Two-dimensional metal-organic framework materials: synthesis, structures, properties and applications. Chem. Rev. 121, 3751–3891 (2021).
López-Cabrelles, J. et al. Isoreticular two-dimensional magnetic coordination polymers prepared through pre-synthetic ligand functionalization. Nat. Chem. 10, 1001–1007 (2018).
Peng, Y. et al. Metal-organic framework nanosheets as building blocks for molecular sieving membranes. Science 346, 1356–1359 (2014).
Liu, Y. et al. Electrochemical synthesis of large area two-dimensional metal-organic framework films on copper anodes. Angew. Chem. Int. Ed. 60, 2887–2891 (2021).
Dong, R. et al. High-mobility band-like charge transport in a semiconducting two-dimensional metal-organic framework. Nat. Mater. 17, 1027–1032 (2018).
Huang, X. et al. A two-dimensional π-d conjugated coordination polymer with extremely high electrical conductivity and ambipolar transport behaviour. Nat. Commun. 6, 7408 (2015).
Duan, J. et al. Ultrathin metal-organic framework array for efficient electrocatalytic water splitting. Nat. Commun. 8, 15341 (2017).
Huang, J. et al. Electrochemical exfoliation of pillared-layer metal-organic framework to boost the oxygen evolution reaction. Angew. Chem. Int. Ed. 57, 4632–4636 (2018).
Zhao, S. et al. Ultrathin metal-organic framework nanosheets for electrocatalytic oxygen evolution. Nat. Energy 1, 1–10 (2016).
Campbell, M. G., Liu, S. F., Swager, T. M. & Dinca, M. Chemiresistive sensor arrays from conductive 2D metal-organic frameworks. J. Am. Chem. Soc. 137, 13780–13783 (2015).
Rodenas, T. et al. Metal-organic framework nanosheets in polymer composite materials for gas separation. Nat. Mater. 14, 48–55 (2015).
Huang, X. et al. Superconductivity in a copper(II)-based coordination polymer with perfect kagome structure. Angew. Chem. Int. Ed. 57, 146–150 (2018).
Liao, W.-M. et al. Tailoring exciton and excimer emission in an exfoliated ultrathin 2D metal-organic framework. Nat. Commun. 9, 2401 (2018).
Yuan, G. et al. Proton-assisted growth of ultra-flat graphene films. Nature 577, 204–208 (2020).
Wang, L. et al. Epitaxial growth of a 100-square-centimetre single-crystal hexagonal boron nitride monolayer on copper. Nature 570, 91–95 (2019).
Lee, Y.-H. et al. Synthesis of large-area MoS2 atomic layers with chemical vapor deposition. Adv. Mater. 24, 2320–2325 (2012).
Cui, X. et al. Rolling up transition metal dichalcogenide nanoscrolls via one drop of ethanol. Nat. Commun. 9, 1301 (2018).
Lin, H. et al. Growth of environmentally stable transition metal selenide films. Nat. Mater. 18, 602–607 (2019).
Stassen, I. et al. Chemical vapour deposition of zeolitic imidazolate framework thin films. Nat. Mater. 15, 304–310 (2016).
Zhang, L., Dong, J. & Ding, F. Strategies, status, and challenges in wafer scale single crystalline two-dimensional materials synthesis. Chem. Rev. 121, 6321–6372 (2021).
Gao, J., Yip, J., Zhao, J., Yakobson, B. I. & Ding, F. Graphene nucleation on transition metal surface: structure transformation and role of the metal step edge. J. Am. Chem. Soc. 133, 5009–5015 (2011).
Dong, J., Ding, D., Jin, C., Liu, Y. & Ding, F. Edge reconstruction-dependent growth kinetics of MoS2. ACS Nano 17, 127–136 (2023).
Howarth, A. J. et al. Best practices for the synthesis, activation, and characterization of metal-organic frameworks. Chem. Mater. 29, 26–39 (2017).
Zhan, G. et al. Observing polymerization in 2D dynamic covalent polymers. Nature 603, 835–840 (2022).
Liu, Y., Huang, Y. & Duan, X. Van der Waals integration before and beyond two-dimensional materials. Nature 567, 323–333 (2019).
Late, D. J. et al. Sensing behavior of atomically thin-layered MoS2 transistors. ACS Nano 7, 4879–4891 (2013).
Song, S. et al. Tuning the stacking modes of ultrathin two-dimensional metal-organic framework nanosheet membranes for highly efficient hydrogen separation. Angew. Chem. Int. Ed. 62, e202312995 (2023).
Acknowledgements
We thank B. Guan and J.L. Yue for the assistance with TEM data analysis. We thank Z.P. Wang for the experimental assistance with GC–MS analysis. We thank Z.J. Zhao for the assistance with XPS data analysis. We thank R.J. Feng for the assistance with FT-IR data analysis. We thank M. Wang for the assistance with the grazing incidence wide-angle X-ray scattering (GIWAXS) analysis. The work was supported by the National Natural Science Foundation of China (22175184 (J.Z.), 22105207 (X.C.)), the CAS Project for Young Scientists in Basic Research (YSBR-053 (J.Z.)), the Strategic Priority Research Programme of the Chinese Academy of Sciences (XDB0520202 (J.Z.)) and the CAS Project for Young Scientists in Interdisciplinary Research (J.Z.).
Author information
Authors and Affiliations
Contributions
J.Z. conceived and designed the research. L.L. and L.H. carried out all syntheses and characterization experiments. P.Z., P.H., C.D. and R.L. assisted with the characterization experiments. G.L. assisted with the GIWAXS measurement and data analysis. Y.Z. and Y.L. assisted with the analysis of the characterization results. X.C. and J.Z. fabricated devices and performed the sensing measurement. J.D. conducted the theoretical analysis on the in-plane growth of MOF. J.Z. supervised the research. J.Z., X.C. and L.L. co-wrote the paper. All authors discussed the results and commented on the manuscript.
Corresponding authors
Ethics declarations
Competing interests
The authors declare no competing interests.
Peer review
Peer review information
Nature Communications thanks Hai Wang and the other, anonymous, reviewer(s) for their contribution to the peer review of this work. A peer review file is available.
Additional information
Publisher’s note Springer Nature remains neutral with regard to jurisdictional claims in published maps and institutional affiliations.
Supplementary information
Source data
Rights and permissions
Open Access This article is licensed under a Creative Commons Attribution 4.0 International License, which permits use, sharing, adaptation, distribution and reproduction in any medium or format, as long as you give appropriate credit to the original author(s) and the source, provide a link to the Creative Commons licence, and indicate if changes were made. The images or other third party material in this article are included in the article’s Creative Commons licence, unless indicated otherwise in a credit line to the material. If material is not included in the article’s Creative Commons licence and your intended use is not permitted by statutory regulation or exceeds the permitted use, you will need to obtain permission directly from the copyright holder. To view a copy of this licence, visit http://creativecommons.org/licenses/by/4.0/.
About this article
Cite this article
Luo, L., Hou, L., Cui, X. et al. Self-condensation-assisted chemical vapour deposition growth of atomically two-dimensional MOF single-crystals. Nat Commun 15, 3618 (2024). https://doi.org/10.1038/s41467-024-48050-5
Received:
Accepted:
Published:
DOI: https://doi.org/10.1038/s41467-024-48050-5
Comments
By submitting a comment you agree to abide by our Terms and Community Guidelines. If you find something abusive or that does not comply with our terms or guidelines please flag it as inappropriate.