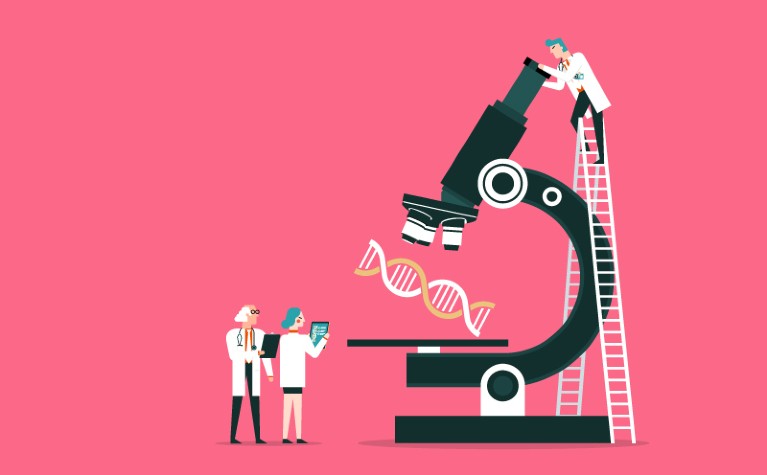
Credit: sorbetto / Getty Images
By the late 1990s, infections caused by serogroup B strains of Neisseria meningitidis (MenB), a major cause of meningococcal meningitis and septicaemia, had resisted all traditional vaccine development efforts. Vaccines for other meningococcal strains, based on their capsular polysaccharides, had been available since the 1960s; however, the capsular polysaccharide of MenB proved to be a poor immunogen owing to its similarity to a human autoantigen. Known antigenic proteins on MenB also proved unsuitable for vaccine development owing to their wide sequence variation.
Following the sequencing of the Haemophilus influenzae genome in 1995 by a team from the Institute for Genomic Research (TIGR), led by Craig Venter, researchers began to consider how sequencing could assist with the development of vaccines for pathogens such as MenB. A collaboration between a team at Chiron Vaccines, led by Rino Rappuoli, the TIGR and Oxford University produced two landmark papers published in Science in 2000, which annotated the whole genome of a MenB strain (MC58) and used this genomic information to identify a large number of novel surface-bound antigens. These reports, authored by Tettelin et al. and Pizza et al., represent the birth of ‘reverse vaccinology’ — the process of developing vaccines using a bottom-up approach from genome to vaccine.
Using the MC58 sequencing data published in Tettelin et al., Pizza et al. used a bioinformatics approach to identify open reading frames (ORFs) in the MC58 genome predicted to have features typical of genes encoding membrane-exposed proteins. A total of 350 candidate ORF sequences were amplified and cloned into expression vectors for His- or glutathione S-transferase (GST)-tagged proteins and expressed in Escherichia coli. Recombinant proteins were then purified and injected into mice, and immune sera from the mice were tested for the presence of specific antibodies through enzyme-linked immunosorbent assay (ELISA) and fluorescence-activated cell sorting (FACS) analysis. Immune sera were also tested for bactericidal activity, with 28 proteins shown to produce bactericidal antibodies — a huge breakthrough considering only five antigens able to induce bactericidal activity had been identified in meningococcal species at that time.
Pizza et al. then analysed the seven ORFs associated with sera that were positive in all of the above assays, comparing these ORFs with genes from other Neisseria strains. Analysis of these ORFs showed they were present in all 31 disease-associated MenB strains tested — and some in other pathogenic Neisseria spp. such as Neisseria gonorrhoeae. Whole-cell ELISA showed that antibodies specific to each of these antigens recognized all 31 of the MenB strains, suggesting that each protein was expressed and exposed at the bacterial surface and therefore accessible to host antibodies. These data therefore identified highly conserved, surface-exposed, immunogenic proteins on MC58. The authors noted that this approach allowed for the screening of antigens that may only have limited immunogenicity in a disease context and thus may be missed by traditional vaccinology approaches.
Following the success of this study, some of the antigens were selected for their capacity to induce broad protection; these antigens included Neisseria heparin binding antigen (NHBA), factor H binding protein (fHbp) and Neisseria adhesin A (NadA). A vaccine was developed consisting of these antigens and the highly variable membrane protein, PorA. After successful clinical studies, the vaccine (4CMenB; Bexsero®) was approved in Europe in 2013, before being introduced into the national immunization programme in the UK in 2015. It is now routinely used for immunization of newborns (less than 12 months of age) in the UK, and is also licensed for immunization of adolescents in the USA.
Since those studies in 2000, the speed of collecting genomic sequences has skyrocketed, and reverse vaccinology approaches have been applied to many other pathogens, including respiratory syncytial virus (RSV), human immunodeficiency virus (HIV), group A and group B Streptococcus species, and antibiotic-resistant strains of Staphylococcus aureus and Streptococcus pneumoniae. A limitation of the technique is that antigens cannot be graded in terms of their predictive ability for immunization; however, recent advances in B cell technologies and structural biology have allowed better characterization of the immunogenicity of antigens identified through reverse vaccinology. The capacity to generate human monoclonal antibodies from memory B cells and plasmablasts, for example, has allowed screening of antigens against human antibodies — improving the assessment and prioritization of bacterial epitopes as vaccine candidates. These advances have led to a new model for rational vaccine design: ‘reverse vaccinology 2.0’.