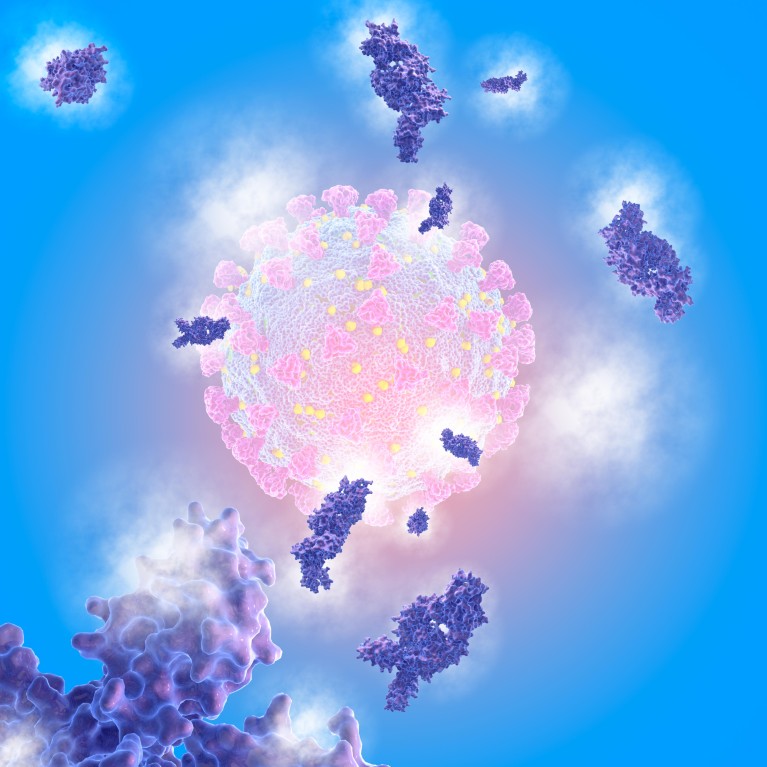
The threat posed by SARS-CoV-2 has accelerated vaccine development, including many using novel technologies.Credit: Mehau Kulyk/SPL/Getty Images
At 6.31am on 8 December 2020, a nurse inserted a needle into Margaret Keenan’s upper left arm. Seconds later, the 90-year-old smiled from behind her mask as onlookers cheered, providing a rare moment of hope at a time when it was in short supply.
The administration of the first dose of an approved COVID-19 vaccine, performed at University Hospital, Coventry, UK, was more than a turning point in the pandemic. It came less than 11 months after the genetic sequence of the SARS-CoV-2 virus was published. Before this, the fastest development was the four years it took for the mumps vaccine in the 1960s.
Keenan’s injection was also the first use, outside a clinical trial, of a messenger RNA (mRNA) vaccine, which can generate an immune response against a virus by providing our cells with the genetic instructions to make part of it. It was, at the last count, one of about 260 COVID-19 candidate vaccines at various stages of development.1 That proliferation of research and development is likely to have implications that reach well beyond the pandemic.
“The vaccine universe is evolving at light speed,” says Emmanuel Hanon, Head of R&D at GSK Vaccines. “To address the emergence of the SARS-CoV2 virus, humanity now has a number of new vaccine technology platforms which can be considered to be a new emerging wave of innovation in vaccinology.”
The roots of a breakthrough
Vaccines rank among the great achievements in medicine. Their use prevents an estimated two to three million deaths per year.2 Many of the vaccines we use today, such as the one for measles, mumps and rubella, are based on use of live, weakened versions of pathogens. Louis Pasteur employed the technique to make vaccines against rabies and cholera in France during the 1880s. Other technologies in use include toxoid vaccines, which consist of harmless versions of toxins produced by pathogens, and subunit vaccines, which trigger immunity by using specific parts of pathogens.
While successful, vaccine development in the twentieth century was based largely on simple testing of many candidate antigens. Little was known about how they activated the immune system that protects us against these pathogens. More recent advances in immunology have based newer vaccine technologies on actual molecular insights into host-pathogen interactions.
In particular, scientists have established a clearer picture of the relationship between generalized immediate innate immunity, and more complex specialized adaptive responses. When it comes to identifying unknown pathogens, for example, dendritic cells are now known to play a central role — patrolling for invaders, capturing antigens and displaying them on their outer surfaces. They activate helper T cells, which in turn trigger B cells, to secrete antibodies, and macrophages to attack pathogens. Helper T cells also direct killer T cells to attack infected cells. Researchers have found that these primary immune responses also trigger the production of long-lasting, antigen-specific memory T and B cells that provide protection against re-infection.
“Our appreciation of the details of how the immune system sits up and takes notice, detecting the presence of pathogen-associated signals, has really come to the fore over the last 10-20 years,” says immunologist, Peter Openshaw, of Imperial College London.
Several advances in synthetic biology helped the creation of next-generation vaccine platforms. The use of a cloned gene into a viral vector for gene therapy3 in 1986, and the achievement of protein expression following the transfer of genes into mouse muscle cells4 in 1990, stimulated interest in the use of genetically engineered genes in vaccines.
Interest started to grow in vaccines consisting of antigen DNA sequences. DNA vaccines were found, in the early 1990s, to be safe and stable, but stimulated disappointingly low immunogenicity in humans5. For some, the answer was to skip the initial stages of DNA transcription by using synthetic mRNA. These were initially unstable and triggered unacceptable reactogenicity by over activating the innate immune system. But researchers found ways to modify mRNAs to silence innate immune responses, and then to protect them within lipid nanoparticles.
Viral vector vaccines are another approach that uses the body’s cells to produce the antigens needed to provoke an immune response. They use viruses, such as adenovirus or measles, modified to prevent them causing disease, to carry the antigen’s genetic instructions into the cell, and are capable of inducing strong immune responses. A vaccine against Ebola became the first viral vector vaccine to gain US and European regulatory approval in 2019.
There has also been important progress in adjuvants, substances that boost vaccine immunogenicity. Aluminium salts have been successfully used since the 1930s; more recently, the hunt for an HIV vaccine stimulated efforts to find new adjuvants.
In the early 1990s, scientists at pharmaceutical company SmithKline Beecham (now part of GSK) carried out tests of combinations of newer adjuvants called MPL, QS-21 and CpG alongside the classical adjuvants. Five of these, called Adjuvant System families, have been investigated in clinical trials, and three are in licensed vaccines today6. Improved immunological understanding means adjuvants can now be selected to enhance specific immune system functions, such as antibody- and/or cell-mediated protection. Many subunit or inactivated COVID-19 candidate vaccines include single or combined adjuvants to help shape immune responses. Adjuvants have been instrumental in enhancing immune responses in previously intractable conditions: in RTS,S, the first vaccine with demonstrated efficacy against malaria in children6, or M72, an investigational vaccine against latent tuberculosis in infected adults7.
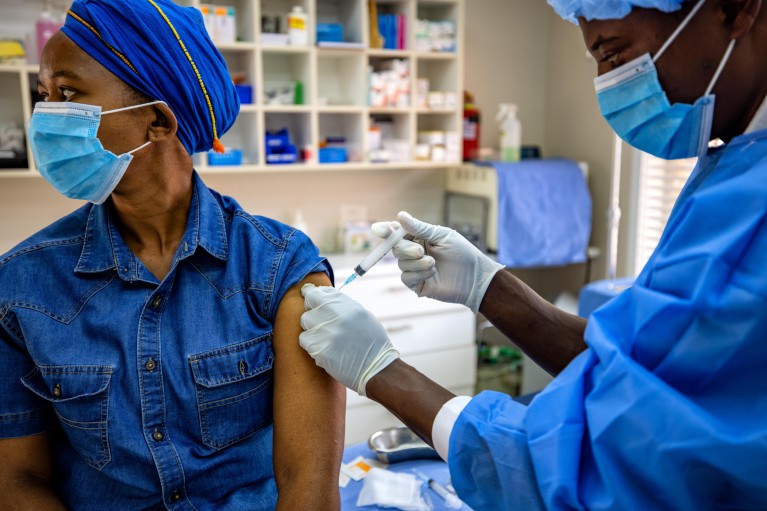
Vaccinations for people in rural communities, particularly in low- or middle-income countries, pose supply-chain challenges.Credit: Martin Harvey/Getty Images
Vaccine technology and COVID-19
Experience with all these technologies underlie the development of COVID-19 vaccines. Many are designed to induce the production of antibodies against the spike proteins on SARS-CoV-2’s surface that latch on to and fuse with human cells. To be effective, these antibodies must target the spike protein in the form it takes before it fuses with host cells, known as its prefusion conformation.
Structural vaccinology is pretty new. In 2013, scientists stabilized the Respiratory Syncytial Virus fusion protein in its prefusion form8; MERS followed in 20159. These works laid the foundation for the rapid publication of the 3D molecular structure of the SARS-CoV-2 spike protein in February 2020 — a milestone in the race to develop COVID-19 vaccines10.
“Structural studies of a number of viruses, such as RSV, have driven our understanding of the importance of this form for cell entry and antibody reactivity,” says immunologist, Diane Griffin, of the Johns Hopkins Bloomberg School of Public Health, in Baltimore, US.
One of the key lessons emerging from COVID-19 is that a variety of different vaccine platforms are needed to deal effectively with different circumstances. mRNA vaccines can induce potent immunological responses and are suitable for specific viral pathogens, but they must be kept at low temperatures. The BioNTech-Pfizer and NIH-Moderna vaccines need to be stored at around –20ºC. This means they can’t be used in parts of the world that lack reliable cold chain systems, for reasons including unreliable electricity supply, lack of transport infrastructure or political instability.
“We know from previous vaccines that the cold chain requirement is an obstacle, particularly in developing countries,” says Griffin. “It’s also possible that as we learn more we find that different sectors of the population respond better to one type or another, so we may need a range of COVID vaccines.”
Many scientists believe tried and trusted technologies still have a major part to play. In 2011, several US institutions teamed up to develop a vaccine to protect against the potential re-emergence of SARS. The group was seeking one that was simple to produce and thermally stable, so that it would be practical for developing countries. They opted for a recombinant protein vaccine, a technology dating back to the 1980s. The result was a yeast-expressed candidate consisting of the receptor-binding domain of the SARS-CoV spike protein.
In early 2020, the collaborating teams tweaked the formula to produce a candidate COVID-19 vaccine called RBD N1C1. Paired with two adjuvants, it will begin a phase III trial in India in April.11 “Novel technologies are important, but in a global pandemic, we do not have the ecosystem to produce them at large enough scale for everyone, and they are expensive,” says microbiologist, Maria Elena Bottazzi, of Baylor College of Medicine, one of those who led the development of RBD N1C1.
Bottazzi and her colleagues are in good company. As of 12 March 2021, 81 SARS-CoV-2 vaccine candidates are in clinical testing,1 including 27 based on recombinant protein technology. DNA, RNA and vector-based vaccines make up another 33. “COVID-19 is dramatically accelerating the use and the validation at large scale of these new technology platforms,” says Hanon. “Post-COVID-19, vaccinology will have changed dramatically.”
Beyond COVID-19
On top of the new technological options, the pandemic is also likely to draw in new talent and resources. “Before COVID-19, vaccines were something of a black box. Now people have a better understanding not only of their value, but also some of the complexities involved in developing them,” says Bottazzi.
Many hope that faster vaccine development will be a positive legacy of the current crisis. The next-generation technologies have significantly shorter development timeframes than traditional approaches. Some practices adopted by regulators in response to COVID-19, such as rolling reviews of trial data and providing advice on the running of parallel clinical trial stages, may streamline processes long-term.
The impact of these advances is not limited to SARS-CoV-2. Prior to the pandemic, researchers had done a lot of work on mRNA cancer vaccines. Validation of the platform for infectious disease could accelerate progress there. The pandemic could also drive advances against other seasonal respiratory viruses. “It would seem quite predictable that we will in the future have common cold, anti-flu and coronavirus vaccines, potentially amalgamated into one shot,” says Openshaw.
COVID-19 is likely to lead to big changes in the vaccines industry. “We are seeing the rapid validation of new technologies at large scale to achieve something in years that would normally take a decade or more,” says Hanon. “There will be new players, winners and losers, and more competition, which is good for the field as it drives creativity.”
Competition is not the only outcome; one of COVID-19’s most important lessons may be quite the opposite. “We’ve seen, during this extraordinary year, companies have been willing to join forces, providing adjuvants for and manufacturing each other’s vaccines,” says Openshaw. “It’s been refreshing to see these new levels of cooperation.”