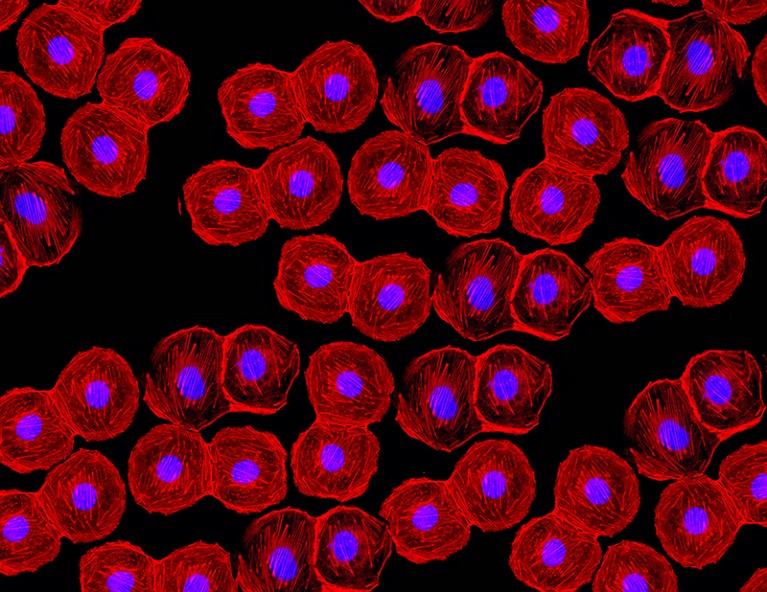
Credit: Edgloris Marys / Alamy Stock Photo
The isolation of the first human embryonic stem cell (hESC) lines in 1998 opened the possibility of stem cell therapies for a variety of conditions. Type 1 diabetes (T1D) is particularly suited to this approach, as transplantation of the insulin-producing pancreatic β-cells could provide long-lasting therapy or even a cure. By the early 2000s, the stem cell differentiation field was benefitting from embryo studies in model organisms that had identified the role of various signalling pathways in controlling pancreatic cell lineage specification.
In 2005, D’Amour et al. reported the production of hESC differentiation cultures containing >80% definitive endoderm cells: the embryonic progenitors of pancreatic cells. Differentiation occurred in 2D culture, using a low serum medium containing activin A, a protein that is essential for endoderm specification in the embryo.
Following this advance, in 2006, D’Amour et al. published a key paper reporting the generation of endocrine pancreatic cells, capable of secreting insulin, glucagon, somatostastin, pancreatic polypeptide and ghrelin from differentiating hESCs. Their differentiation protocol included five stages, during which hESCs went through a series of intermediate cellular states: definitive endoderm, primitive gut tube, posterior foregut, pancreatic endoderm and endocrine precursors and hormone-expressing endocrine cells. Specific growth factors were supplied at each step, in an effort to mimic embryonic pancreas development. Indeed, characterization of mRNA and proteins in the different cell states showed that they resembled the equivalent embryonic endodermal progenitors.
At stage five of this study, staining by zinc-chelating agent dithizone was used to identify the pancreatic endocrine cells. Their insulin content was similar to that of primary adult human islets and the de novo synthesis of insulin was confirmed by mRNA, C-peptide and pro-insulin protein measurement. However, compared with adult islets, the C-peptide content of hESC-derived cells was inferior, indicating a reduced efficiency in processing pro-insulin. C-peptide secretion was responsive to multiple secretory stimuli, albeit only minimally responsive to glucose.
Subsequently, in 2008, the same group (Kroon et al.) succeeded in generating glucose-responsive endocrine cells in vivo, following transplantation of hESC-derived stage-four pancreatic endoderm in mice. These cells expressed key β-cell transcription factors, showed efficient processing of pro-insulin and contained mature secretory granules. Importantly, the hESC-derived endocrine cells protected the mice against hyperglycaemia.
In 2014, Rezania et al. described a seven-stage hESC differentiation protocol that generated glucose-responsive insulin-producing β-cells that reversed diabetes in mice upon transplantation. In the same year, Pagliuca et al. reported the in vitro large-scale generation of glucose-responsive, insulin-expressing β-cells derived from human pluripotent stem cells (hPSCs), by sequentially modulating key signalling pathways in a 3D culture system. These hPSC-derived β-cells were very similar to human β-cells in terms of gene expression and ultrastructure and also ameliorated hyperglycaemia in diabetic mice upon transplantation.
In 2015, Russ et al. reported a simplified suspension-based, directed differentiation protocol to generate glucose-responsive insulin-producing human β-cells from hPSCs in vitro and in vivo. The resulting cells reduced blood levels of glucose in diabetic mice in a matter of weeks, as opposed to months in previous studies.
In 2019, Nair et al. reported the generation in vitro of mature β-cells from hESCs, by allowing the immature β-like cells to form islet-like clusters, thus recapitulating endocrine cell clustering in vivo. Mature β-cells showed enhanced metabolic and structural maturation of mitochondria and hyperglycaemia was reduced within days of transplantation in diabetic mice.
In 2020, Hogrebe et al. discovered that the state of actin polymerization influences pancreatic differentiation in vitro. Thus, timed actin depolymerization during differentiation led to the generation of β-cells from hPSCs that rapidly reversed diabetes in mice and maintained normoglycaemia for 9 months.
Fifteen years after the first insulin-secreting cells were generated by differentiating hESCs in vitro, we still do not have a β-cell replacement therapy for T1D. However, thanks to the studies mentioned here, and many others, we are much closer to this goal. Apart from further optimizing the efficiency and scalability of mature β-cell production, important issues, such as shielding the transplanted stem-cell-derived β-cells from immune rejection and ensuring their purity, need to be resolved before a stem-cell-based therapy becomes a reality.